38.3: Impacts on the cryosphere and biosphere
- Page ID
- 22836
\( \newcommand{\vecs}[1]{\overset { \scriptstyle \rightharpoonup} {\mathbf{#1}} } \)
\( \newcommand{\vecd}[1]{\overset{-\!-\!\rightharpoonup}{\vphantom{a}\smash {#1}}} \)
\( \newcommand{\id}{\mathrm{id}}\) \( \newcommand{\Span}{\mathrm{span}}\)
( \newcommand{\kernel}{\mathrm{null}\,}\) \( \newcommand{\range}{\mathrm{range}\,}\)
\( \newcommand{\RealPart}{\mathrm{Re}}\) \( \newcommand{\ImaginaryPart}{\mathrm{Im}}\)
\( \newcommand{\Argument}{\mathrm{Arg}}\) \( \newcommand{\norm}[1]{\| #1 \|}\)
\( \newcommand{\inner}[2]{\langle #1, #2 \rangle}\)
\( \newcommand{\Span}{\mathrm{span}}\)
\( \newcommand{\id}{\mathrm{id}}\)
\( \newcommand{\Span}{\mathrm{span}}\)
\( \newcommand{\kernel}{\mathrm{null}\,}\)
\( \newcommand{\range}{\mathrm{range}\,}\)
\( \newcommand{\RealPart}{\mathrm{Re}}\)
\( \newcommand{\ImaginaryPart}{\mathrm{Im}}\)
\( \newcommand{\Argument}{\mathrm{Arg}}\)
\( \newcommand{\norm}[1]{\| #1 \|}\)
\( \newcommand{\inner}[2]{\langle #1, #2 \rangle}\)
\( \newcommand{\Span}{\mathrm{span}}\) \( \newcommand{\AA}{\unicode[.8,0]{x212B}}\)
\( \newcommand{\vectorA}[1]{\vec{#1}} % arrow\)
\( \newcommand{\vectorAt}[1]{\vec{\text{#1}}} % arrow\)
\( \newcommand{\vectorB}[1]{\overset { \scriptstyle \rightharpoonup} {\mathbf{#1}} } \)
\( \newcommand{\vectorC}[1]{\textbf{#1}} \)
\( \newcommand{\vectorD}[1]{\overrightarrow{#1}} \)
\( \newcommand{\vectorDt}[1]{\overrightarrow{\text{#1}}} \)
\( \newcommand{\vectE}[1]{\overset{-\!-\!\rightharpoonup}{\vphantom{a}\smash{\mathbf {#1}}}} \)
\( \newcommand{\vecs}[1]{\overset { \scriptstyle \rightharpoonup} {\mathbf{#1}} } \)
\( \newcommand{\vecd}[1]{\overset{-\!-\!\rightharpoonup}{\vphantom{a}\smash {#1}}} \)
\(\newcommand{\avec}{\mathbf a}\) \(\newcommand{\bvec}{\mathbf b}\) \(\newcommand{\cvec}{\mathbf c}\) \(\newcommand{\dvec}{\mathbf d}\) \(\newcommand{\dtil}{\widetilde{\mathbf d}}\) \(\newcommand{\evec}{\mathbf e}\) \(\newcommand{\fvec}{\mathbf f}\) \(\newcommand{\nvec}{\mathbf n}\) \(\newcommand{\pvec}{\mathbf p}\) \(\newcommand{\qvec}{\mathbf q}\) \(\newcommand{\svec}{\mathbf s}\) \(\newcommand{\tvec}{\mathbf t}\) \(\newcommand{\uvec}{\mathbf u}\) \(\newcommand{\vvec}{\mathbf v}\) \(\newcommand{\wvec}{\mathbf w}\) \(\newcommand{\xvec}{\mathbf x}\) \(\newcommand{\yvec}{\mathbf y}\) \(\newcommand{\zvec}{\mathbf z}\) \(\newcommand{\rvec}{\mathbf r}\) \(\newcommand{\mvec}{\mathbf m}\) \(\newcommand{\zerovec}{\mathbf 0}\) \(\newcommand{\onevec}{\mathbf 1}\) \(\newcommand{\real}{\mathbb R}\) \(\newcommand{\twovec}[2]{\left[\begin{array}{r}#1 \\ #2 \end{array}\right]}\) \(\newcommand{\ctwovec}[2]{\left[\begin{array}{c}#1 \\ #2 \end{array}\right]}\) \(\newcommand{\threevec}[3]{\left[\begin{array}{r}#1 \\ #2 \\ #3 \end{array}\right]}\) \(\newcommand{\cthreevec}[3]{\left[\begin{array}{c}#1 \\ #2 \\ #3 \end{array}\right]}\) \(\newcommand{\fourvec}[4]{\left[\begin{array}{r}#1 \\ #2 \\ #3 \\ #4 \end{array}\right]}\) \(\newcommand{\cfourvec}[4]{\left[\begin{array}{c}#1 \\ #2 \\ #3 \\ #4 \end{array}\right]}\) \(\newcommand{\fivevec}[5]{\left[\begin{array}{r}#1 \\ #2 \\ #3 \\ #4 \\ #5 \\ \end{array}\right]}\) \(\newcommand{\cfivevec}[5]{\left[\begin{array}{c}#1 \\ #2 \\ #3 \\ #4 \\ #5 \\ \end{array}\right]}\) \(\newcommand{\mattwo}[4]{\left[\begin{array}{rr}#1 \amp #2 \\ #3 \amp #4 \\ \end{array}\right]}\) \(\newcommand{\laspan}[1]{\text{Span}\{#1\}}\) \(\newcommand{\bcal}{\cal B}\) \(\newcommand{\ccal}{\cal C}\) \(\newcommand{\scal}{\cal S}\) \(\newcommand{\wcal}{\cal W}\) \(\newcommand{\ecal}{\cal E}\) \(\newcommand{\coords}[2]{\left\{#1\right\}_{#2}}\) \(\newcommand{\gray}[1]{\color{gray}{#1}}\) \(\newcommand{\lgray}[1]{\color{lightgray}{#1}}\) \(\newcommand{\rank}{\operatorname{rank}}\) \(\newcommand{\row}{\text{Row}}\) \(\newcommand{\col}{\text{Col}}\) \(\renewcommand{\row}{\text{Row}}\) \(\newcommand{\nul}{\text{Nul}}\) \(\newcommand{\var}{\text{Var}}\) \(\newcommand{\corr}{\text{corr}}\) \(\newcommand{\len}[1]{\left|#1\right|}\) \(\newcommand{\bbar}{\overline{\bvec}}\) \(\newcommand{\bhat}{\widehat{\bvec}}\) \(\newcommand{\bperp}{\bvec^\perp}\) \(\newcommand{\xhat}{\widehat{\xvec}}\) \(\newcommand{\vhat}{\widehat{\vvec}}\) \(\newcommand{\uhat}{\widehat{\uvec}}\) \(\newcommand{\what}{\widehat{\wvec}}\) \(\newcommand{\Sighat}{\widehat{\Sigma}}\) \(\newcommand{\lt}{<}\) \(\newcommand{\gt}{>}\) \(\newcommand{\amp}{&}\) \(\definecolor{fillinmathshade}{gray}{0.9}\)Let us consider the impacts of carbon burial on three phenomena: fire, climate, and animal physiology.
Fire

We listed a few examples of anthropogenic fire above, but fire is also a natural part of the modern Earth system. Wildfires can be sparked by lightning strikes or volcanic eruptions. They are more likely to ignite and to spread in areas that are temporarily warm and dry, but have seen wet enough conditions in the past to build up a supply of plant fuel. Combustion occurs when the plant tissues react with atmospheric oxygen, and release the carbon in the plants back into the atmosphere as \(\ce{CO2}\).
So oxygen is a pre-requisite for wildfire, and oxygen levels have changed throughout Earth history. How much oxygen is necessary? It turns out that fire requires at least 13% \(\bf{\ce{O2}}\) in the surrounding atmosphere. Over the long-term, our planet has bulked up the free oxygen content of its atmosphere, from an initial 0% to the current ~21%. It is estimated that the critical 13% threshold was crossed in the Silurian. Two lines of evidence support this interpretation:
1. The oldest terrestrial plant fossils are spores that date to the Ordovician, suggesting colonization of the land surface by organisms akin to club mosses. These plants provided fuel to burn, but also shelter for animals. The oldest terrestrial animal fossils date to shortly thereafter. As plants colonized the land, they led to more of the planet’s surface being devoted to (incidental) oxygen production. It is thought that oxygenation of the atmosphere would also have led to the development of an ozone (\(\ce{O3}\)) layer, which would have enabled animals to spread across the land due to lowering the influx of harmful ultraviolet radiation from the Sun.

Figure \(\PageIndex{2}\): Charcoal clasts in Silurian sandstone from Wales. (Modified from Figure 3 of Glasspool & Gastaldo (2022).)
2. By 420 Ma (Silurian), charcoal starts showing up. Charcoal is a carbon-rich version of what used to be wood, but all the water and volatile components have been driven off through heating it in the presence of limited amounts of oxygen. It is our first evidence that fires had started burning on Earth.
With the exception of a “gap” in the mid-Devonian, charcoal has been a common constituent of terrestrial sediments for the rest of the Phanerozoic Eon. Thanks to the rise in oxygen levels, fire has become a standard phenomenon on the surface of Earth. So the burial of plant biomass preserves some carbon for the future, but leaves behind oxygen at the surface, and that oxygen that makes it more likely for unburied plants to burn.
Ice
The greenhouse effect needs a quick recap in order to understand what happens next.

\(\ce{CO2}\) is an invisible gas. But it’s only invisible to the visible wavelengths of light. Under other wavelengths of light, it shows up as a dense foggy cloud. Specifically, infrared light cannot pierce through \(\ce{CO2}\) – the infrared light’s energy is absorbed and re-radiated in a random direction. Examine the cartoon illustration of this idea at right: When a beam of visible light is directed through a glass box containing \(\ce{CO2}\), it all passes through to the other side. But when infrared light is shone through instead, it gets stopped, and so less than 100% makes it across to the far side of the box. So \(\ce{CO2}\) is selectively transparent to different wavelengths of light.
This matters intensely for the regulation of Earth’s climate. Though the planet is irradiated by visible wavelengths of light, Earth materials absorb that light, and warm up. They then re-radiate some of the energy in the infrared wavelengths. Hot objects, like the pavement of a street on a summer’s day, give off a lot of infrared radiation. If this infrared light were unimpeded, the hot street would shoot it straight out into space. But thankfully, that isn’t what happens. Instead, greenhouse gases like \(\ce{CO2}\) catch some of those infrared photons and spit them back out again. As a result, Earth doesn’t drop to the frigid temperatures of outer space every night. If you want a planet where liquid water can exist, you need to equip your planet with greenhouse gases to modulate its temperature. Greenhouse gases like \(\ce{CO2}\) are therefore a prerequisite for life.
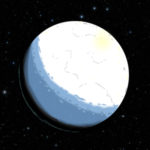
\(\ce{CO2}\) is a tiny proportion of our atmosphere by volume (we measure its presence in parts per million, not percent or even parts per thousand), but it is very, very important for regulating heat loss. Please do not confuse “small amount by volume” with “unimportant.” Because of the way atmospheric \(\ce{CO2}\) interacts with infrared light, its level is the main Earth-derived control on climate.
Now let’s think about how planetary-scale carbon burial impacts the greenhouse effect.
In the mid- to late-Paleozoic, plants evolved and spread across the land area of our planet. These plants extracted \(\ce{CO2}\) from the atmosphere, but if they burned or rotted over short timescales, that carbon went straight back into the atmospheric reservoir as \(\ce{CO2}\). That’s an example of the short-term carbon cycle. On the other hand, if the plants did their photosynthesis, captured the carbon from the \(\ce{CO2}\) and flung out \(\ce{O2}\) as a waste product, and then the plants were buried in swampy sediments, then the plant carbon no longer can interact with the oxygen in the atmosphere. It has been sequestered. Now we’re discussing the long-term carbon cycle.

There is evidence of the reduction of atmospheric \(\ce{CO2}\) at several points in Earth history. During the late Devonian period, excessive formation of black shale (full of carbon captured by marine plankton) led to an ice age and one of the big five mass extinctions. During the ensuing Carboniferous period, repeated transgressive burials of coastal swamps led to cyclothems including the coal seams that powered the Industrial Revolution. The Carboniferous gets its name from the carbon-rich nature of the rocks laid down at that time in England. Please realize that all that carbon in the rock is carbon that wasn’t in the atmosphere. In the Neoproterozoic Era, the planet froze over when \(\ce{CO2}\) levels got zeroed out due to excessive weathering of the continental crust. It wasn’t organic carbon burial in that case, but reaction of \(\ce{CO2}\) with silicate minerals that caused the \(\ce{CO2}\) drawdown and cooling.
In summary, high rates of carbon burial lead to lower \(\ce{CO2}\) levels, which causes a reduction in the greenhouse effect, and thus global cooling. Sometimes this global cooling is severe enough to cause a mass extinction.
Big bugs
But burial of lots of organic carbon doesn’t just lower global \(\ce{CO2}\) levels. It also means that the waste product of photosynthesis builds up and has a lesser amount of carbon available to react with. That waste product, of course, is \(\ce{O2}\). With less organic carbon available for oxidation, atmospheric oxygen levels would be expected to go up.
In the planet’s earliest years, there was no free oxygen in its atmosphere. Gradually it built up through time, largely thanks to the photosynthetic work of cyanobacteria. By the Paleoproterozoic/ Mesoproterozoic boundary, it had accumulated to something like 1% of the atmosphere by volume. This has been dubbed the Great Oxygenation “Event.” Currently, our atmosphere has an atmosphere that consists mainly of nitrogen (\(\ce{N2}\)) but also includes ~21% free oxygen (\(\ce{O2}\)).

However, it hasn’t been a straight path from the GOE to our present oxygenation. During the Carboniferous period, rates of carbon burial were high, so that means atmospheric \(\ce{CO2}\) was low and therefore atmospheric \(\ce{O2}\) was high. Estimates are that it got as high as 35% of the atmosphere. In such an atmosphere, you wouldn’t have to inflate your lungs as often in order to get the oxygen you need.
Insects don’t have lungs. Instead, they have a network of branching tubes that runs throughout their body, and through which air circulates. The maximum size of insects today is controlled by the efficiency of this tube network in delivering sufficient oxygen to the tissues that need it. The Goliath beetle is the current record-holder as “biggest bug.” It can get up to 4.5 inches (11 cm) long. While that’s certainly impressive, it doesn’t hold a candle to this:

That’s a dragonfly the size of a hawk. Its wingspan is 28 inches (70 cm). It could never survive today, but during the Carboniferous, with an atmosphere of 35% oxygen, such a huge insect was plausible. Other examples of giant arthropods include Arthopleura and Megarachne.