4.5: Weathering
- Last updated
- Jul 10, 2023
- Save as PDF
- Page ID
- 22339
( \newcommand{\kernel}{\mathrm{null}\,}\)
2.5.1 Weathering Introduction
Weathering is the term used for the chemical decomposition and physical disintegration of bedrock at and just below the earth’s surface. Weathering acts upon all bedrock near the surface, although with greatly varying nature and rate depending upon a number of factors.
Rock at depth tends to be brought upward toward the surface, by gradual unroofing by weathering at the surface and removal of the weathering products. It’s a matter of relative motion: the bedrock at depth may be either motionless (relative to, say, the center of the earth) or moving slowly upward by uplift or downward by subsidence, but in any case it’s moving upward relative to the earth’s surface if material at the surface is being stripped away. Astherock nears the surface, it tends to be subjected to what’s called near-surface alteration: it finds itself at much lower pressures and temperatures than at greater depth, and surface-derived waters, which tend to be oxygenated and carry dissolved CO2, have the opportunity to cause certain chemical reactions in many constituent minerals, like ferromagnesian silicates and feldspars. In a sense, you can think of such near-surface alteration as a precursor or forerunner to weathering in the stricter sense. But despite such near-surface alteration, the rock is still solidly bedrock, and nowhere near a condition that could be described as regolith.
Weathering, in the usually accepted sense, acts upon rock that is close enough to the surface to be affected not only by active circulation of surface- derived waters but also by such things as temperature changes and plant growth. But in thinking about weathering you don’t have to restrict yourself to the actual rock surface, upon which the sun shines!
Weathering is traditionally subdivided into two sets of processes:
- chemical weathering, the chemical decomposition of some or all of the constituent minerals or the bedrock.
- physical weathering, the mechanical breakdown or disintegration of the bedrock itself into particles large and small.
This is a useful distinction, but here’s a very important fact to understand: chemical weathering and physical weathering tend to act simultaneously and reinforce one another.
2.5.2 Chemical Weathering
Chemically, the earth’s surface is a very reactive place. To begin with, in most places and at least at certain times the surface is an aqueous environment, and water is not only a very effective solvent in itself but also a necessary medium for a great many chemical reactions.
Owing to the high content of oxygen in the atmosphere, in most places the earth’s surface is a well oxygenated environment, as in the shallow subsurface wherever the concentration of organic matter is not so great and the permeability so low (as is common in bogs and swamps) that the oxygen is consumed in oxidation of organic matter before it passes downward to deeper depths. (If you’re unsure about the nature of oxidation, there will be some background material on it later.) Because so many of the common rock-forming minerals contain ferrous iron (that is, iron in the reduced state, and thus susceptible to oxidation to the insoluble ferric state), oxidation reactions are an important aspect of chemical weathering.
In the same way, because the atmosphere contains a substantial percentage of carbon dioxide (CO2), surface waters invariably contain appreciable dissolved CO2. You know already that CO2 readily dissolves in water. That dissolved CO2 reacts with the water itself to form a weak acid, carbonic acid, according to the reaction
CO2+H2O−⇀↽−H2CO3
At equilibrium, about one percent of the dissolved CO2 is in the form of carbonic acid. Most minerals are less stable in an acidic medium than in a neutral medium, so the dissolved CO2 enhances the weatherability of many minerals.
Specifically, what’s going on with Reaction 1? A molecule of carbon dioxide, which consists of a carbon atom tightly bound up with two oxygen atoms, combines with a molecule of water, which consists of an oxygen atom tightly bound up with two hydrogen atoms, in such a way that a single, new, larger molecule, with the chemical formula H2CO3, is produced. In the aqueous solution, this process is going on all the time, and at the same time the H2CO3 molecules are being changed back into CO2 and H2O molecules. Where the balance is struck depends on the imposed concentration of CO2, which in this case comes either from the atmosphere or from the chemical breakdown of organic matter. (You probably know that, by photosynthesis, plants take up water and carbon dioxide and convert it into complex carbohydrate molecules, and when the plant dies and the plant tissues are exposed to an oxygenated aqueous environment the reverse happens and the organic matter is broken back down into carbon dioxide and water.)
Carbonic acid is not the only acid common in natural near-surface waters: in most land areas, organisms (both plants and animals) living on or in the soil (that is, the uppermost part of the layer of regolith) use carbon dioxide and various nutrients to produce organic matter, which in its decay back to the constituent compounds tends to produce a variety of weak organic acids.
In the rest of this section, several kinds of chemical reaction will show the range of weathering reactions that are important in chemical decomposition of bedrock minerals. They involve solution, oxidation, and carbonation, in various ways. Keep in mind that although these particular reactions are important, they are just representative reactions: details vary depending upon the assemblage of minerals that are being weathered, and upon their particular compositions (which, remember, vary widely because of the wide range of ionic substitutions in silicate minerals).
Solution
Some minerals simply dissolve in water. Halite (sodium chloride) and gypsum (hydrated calcium sulfate) are good examples:
NaCl(s)−⇀↽−Na++Cl−
CaSO4⋅H2O−⇀↽−Ca2++SO2−4+H2O
This is the simplest kind of weathering reaction to understand, but not the most important. A more important, and more complicated, kind of solution involves calcite, the constituent of limestone:
CaCO3(s)+CO2+H2O−⇀↽−Ca2++2H++CO2−3
The reason this reaction is more complicated is that it involves the atmospheric carbon dioxide dissolved in the water. The CO2 has to be involved because of the CO32- ion both in the structure of the mineral and as a product of the solution and then dissociation of CO2 in water. (Incidental note: this reaction can go in either direction, depending upon the concentration of CO2: if CO2 is added to the system, as in weathering environments, calcite is dissolved; if it’s extracted from the system, as in certain shallow warm ocean environments, calcium carbonate sediment is precipitated. Think about that the next time you’re lying on a white carbonate-sand beach in the Caribbean.)
carbonation of silicate minerals that don’t contain aluminum or iron:
Most silicate minerals that don’t contain aluminum contain both Fe2+ and Mg2+ ions in various proportions. Rather than writing a single weathering reaction for such minerals, it’s neater to look at separate reactions for those with only magnesium and those with only iron. Such minerals, although they exist, are not common, but their weathering reactions are instructive. First look at the weathering of olivine, which is a common mineral in igneous rocks, and one that’s very susceptible to chemical weathering. Most olivines in igneous rocks contain some iron in addition to magnesium, but let’s assume, for simplicity, that we are dealing with a pure magnesium olivine:
Mg2SiO4+4CO2+4H2O→2Mg+2+4HCO−3+H4SiO4
(I’ve used the single arrow to the right, rather than the double arrow, here and below because the reverse of these reactions is not actually how the minerals get built under deep Earth conditions.)
Note that “silica” (that is, SiO2 in some form or other) is extracted from the original silicate mineral. In most natural waters, the dominant species of silica in solution is H4SiO4, a weak acid called silicic acid. It’s in the form of a molecule that consists of four hydrogen atoms, four oxygen atoms, and one silicon atom, all tightly bonded together.
carbonation of silicate minerals that don’t contain aluminum but do contain iron:
Now look at a ferromagnesian mineral with all Fe and no Mg. (We’ll assume that the mineral is a pyroxene mineral; no details here.) In our oxygen- rich near-surface environment, it’s a two-step process. The first step is like that expressed in Reaction 5 above, but the numerical values of the coefficients in front of the reactants and products are somewhat different, because we started out with a mineral with a different chemical formula. Qualitatively, the result is the same: the iron-bearing silicate mineral is broken down in the presence of water and carbon dioxide to put iron and silica in solution. The dissolved iron is in the ferrous (“plus-two”) form, which is freely soluble in pure water. It is converted in the presence of oxygen to ferric iron oxide and silica in solution:
FeSiO3+2CO2+3H2O→Fe+2+2HCO−3+H4SiO4
But that’s not the end of the story, because the ferrous iron in solution reacts with
oxygen dissolved in the water, to precipitate an iron oxide mineral, Fe2O3:
2Fe+2+3O2→2Fe2O3
The iron in the Fe2O3 mineral is in the ferric (“plus-three”) form, which is almost totally insoluble in pure water.
The representation on Reaction 7 is still a bit of a fake, however, because ferric oxides produced at the earth’s surface are commonly hydrous or hydrated: one could describe them as hydrous ferric oxides. There are several closely related minerals of that kind; the most common are goethite, FeO(OH), and ferrihydrite, 5Fe2O3.9H2O). They all have colors that range from yellow through orange to brown; that’s why weathered surfaces on bedrock are so commonly of those colors. In a sense, ferrous-iron-bearing rocks rust when exposed at the earth’s surface.
If we had started with a real pyroxene, with a combination of Fe2+ ions, Mg2+ ions, and Ca2+ ions, the latter two would have been freed to solution while the first would have found its way into insoluble oxides; that’s because magnesium and calcium are readily soluble in water but ferric iron is almost completely insoluble.
weathering of aluminosilicates:
Now to the biggest show of all in chemical weathering. The example here will be what is certainly the single most important weathering reaction on earth: hydrolysis and carbonation of feldspar. We’ll use potassium feldspar as the example; weathering of plagioclase feldspar is similar.
2KAlSi3O8+2H2CO3+9H2O→Al2Si2O25(OH)4+4H4SiO4+2K++2HCO−3
Comments:
- The first product on the right is the clay mineral kaolinite. (There will be more material on clay minerals in a later section.) Other kinds of clay minerals are possible as solid products of weathering of feldspar as well, depending upon the details of the chemical environment.
- The products in solution are silicic acid, as before, and potassium ions.
- If the feldspar had been plagioclase, Na+ and Ca2+ ions would have gone into solution rather than K+ ions.
- The reaction could have been rewritten slightly to start with CO2 rather than with H2CO3. The differences are immaterial. Those of you who are well versed in chemistry can try to rewrite the reaction by adding to it the following reaction: 2CO2 + 2H2O ⇔ 2H2CO3.
In conclusion, if you don’t remember anything else about chemical weathering, remember this:
when feldspar is weathered, by reaction with water and carbon dioxide, the result is a clay mineral, positive ions (K+ and/or Na+ and/or Ca2+) in solution, and silica, mostly in the form of H4SiO4, in solution.
In intense weathering situations, in warm and humid environments, weathering of aluminosilicate minerals like feldspar can go a step further, to the ultimate weathering product:
2Al2Si2O25(OH)4→4Al(OH)3+4H4SiO4+5H2O
The kaolinite, which itself was the weathering product of potassium feldspar, is stripped further of all of its silica, to produce a totally insoluble aluminum hydroxide mineral and more silica in solution. This is the ultimate weathering residue! There are several hydrous aluminum oxide minerals that come out of a reaction like this; the mineral gibbsite is the most common. Surficial materials that are especially rich in such insoluble hydrous aluminum oxides are called bauxite. As you probably know, such weathering-residue mixtures of hydrous aluminum oxides are the world’s supply of aluminum ore. Depending upon the composition of the original rocks, such ultimate weathering residues are commonly mixed with insoluble hydrous iron oxides as well.
One final point, which will be important for our later consideration of sediments and soils: all of the solid weathering products in the reactions above— clay minerals and various oxides of aluminum and ferric iron—are commonly of finer particle size than the minerals from which they are produced during weathering.
What are the controls on chemical weathering? Viewed on the large scale, there are four major controls on chemical weathering:
- climate: The intensity of chemical weathering increases with precipitation and temperature. The reason it increases with precipitation is that weathering requires an aqueous medium for the reactions. The greater the percentage of time a given mass of weatherable rock is saturated with surface water, the more chemical weathering it is likely to experience. The reason that the intensity of chemical weathering increases with temperature is that, as with all chemical reactions, the rate of the reaction increases with temperature. That’s basically because the vigor of the thermal agitation of the atoms and molecules increases with temperature.
- rock type: Some kinds of rocks are more susceptible to chemical weathering than others. Rocks that contain minerals that were formed under conditions of the high temperatures and pressures of the deep crust or upper mantle are much more weatherable than rocks that contain abundant minerals like quartz, calcite, or muscovite, which are stable, or at least only slowly weatherable, under most Earth-surface conditions. For that reason, most igneous and metamorphic rocks are generally more susdceptible to weathering than most sedimentary rocks.
- relief: Regions with high relief usually also have steep slopes. Gravity-driven mass movements (more on that in a later chapter) then are effective at stripping away newly weathered material, thereby exposing fresher bedrock to continued chemical weathering. In regions with low relief, however, the layer of regolith thickens to the point where the underlying bedrock is largely sealed off from further contact with the surface environment of chemical weathering.
- vegetation: As mentioned earlier, when plant materials decay they release carbon dioxide and weak acids into the regolith environment. These substances acidify the surficial material, thereby tending to drive the various chemical weathering reactions discussed above to the right.
There’s another way of looking at the controls on chemical weathering: from the standpoint of what a small mass of weatherable material actually senses. This is the small-scale view, in contrast to the foregoing largescale view. It boils down, largely, to four more specific physicochemical factors:
- mineral composition of the rock
- chemical composition of the water
- rate of passage of water through the rock • temperature
2.5.3 Physical Weathering
Most rocks are strong: you need to pound on them with a hammer to break them. The strongest rocks, like tough quartzites, yield only reluctantly to even the most vigorous hammer blows. Other rocks, like soft sandstones, you can break with your own hands. (In fact, there is a continuous gradation between loose and unconsolidated sediment and strongly lithified sedimentary rock, depending in large part upon depth of burial.) Rock strength is a complicated topic, which is important for the construction industry, as well as for geoscientists who try to understand rock fracture that causes large earthquakes.
Let me a bit more specific about the nature of the strength of solids like rocks. There are three ways of breaking solids: by compression, by tension, and by shear (Figure 2-5). In rocks, the compressive strength and the shear strength are both large, but the tensile strength is much less: generally, it’s much easier to break a rock by pulling it apart than by squeezing it. (That’s true of concrete, an artificial rock, as well.)

The easiest way to break a rock is to wedge open a preexisting crack. That’s partly because of the low tensile strength, but also because of an effect known as stress concentration at the tip of the propagating crack (for reasons that are beyond the scope of this course). A simple analogy can serve well here: it’s much easier to “break” a sheet of paper in tension by starting a cut and then pulling the edges of the cut apart than by grasping the sheet at opposite edges and pulling on it uniformly. When a rock fractures in tension, either rapidly as a result of a blow or an explosion, or slowly, by a variety of natural weathering processes, the crack enlarges as the tip of the crack propagates into the non-fractured rock.
Breakage of polycrystalline aggregates like rocks (or metals) is more complicated than breakage of homogeneous materials like glass. That’s because the crystal grains of the rock are joined together at the grain boundaries, so the strength of the grain contacts needs to be taken into consideration as well as the strength of the mineral grains themselves. When a rock is stressed, extremely fine cracks tend to develop first along the grain boundaries.
Rocks at the surface tend to be broken down (disaggregated, disintegrated) by a variety of processes, whose importance varies greatly from place to place and from time to time. These physical processes (you might also call them mechanical processes) have traditionally been cited as important in physical weathering, but definitive quantitative studies of the various effects are discouragingly scarce. I’ll set them out for you here without making much of an attempt to rank them in importance, except for some concluding comments at the end of this section.
sheeting: Sheeting is a style of rock fracturing that develops sets of fractures just below the bedrock surface and concordant with it. The reason such fractures tend to form is that as rock is brought up to the surface it is unloaded: the weight of overlying rock is reduced. The rock tries to expand slightly (rock has small but definite compressibility), and it can expand only upward, not sideways. This leads to development of fractures that are roughly parallel with the rock surface. Sheeting is most common in otherwise massive and non-fractured bedrock. The sheeting joints decreases rapidly in spacing downward from the surface, usually disappearing within several meters. The next time you see a photo of the mountain slopes in Yosemite, think in terms of sheeting fractures.
exfoliation: Exfoliation (Figure 2-6) is the separation, during weathering, of successive shells, a few millimeters to some tens of centimeters thick, from massive bedrock. The mechanical effect is somewhat like that of sheeting: as the thin near-surface layer of bedrock undergoes chemical weathering, the weathering-product minerals tend to be of lower density that the original minerals, which means larger volume, so the thin uppermost zone of the rock tries to expand. It can expand only upward, so fractures parallel to the rock surface are formed. In this situation, chemical weathering and mechanical weathering act together to disintegrate the bedrock. A related process leads to what’s called spheroidal weathering: the exfoliation and spalling is slightly more effective at edges and corners than on rock faces, so in an outcrop cut by sets of planar fractures in various orientations (such fractures are called joints; they are ubiquitous in bedrock outcrops) the edges and corners are rounded off to produce a very spheroidal or “blobby” look to the outcrop. In advanced cases the spheroids are completely freed from the outcrop, and lie around on the surface or roll downslope.

granular disintegration: Granular disintegration is another process that is in part a mechanical effect of chemical weathering. Many if not most rocks consist of a mixture of minerals, some susceptible to chemical weathering and others not. As the weatherable minerals expand upon incipient chemical weathering, while the non-weatherable minerals retain their original size, intergranular forces are set up, which tend to break the bonds between mineral grains. The mineral grains fall from the rock surface, one by one, to leave an overall smoothly rounded but granular-rough surface. The mass of loose grains at the base of the outcrop is called grus. This kind of weathering is most common in crystalline rocks like granite.
thermal expansion and contraction: Expansion and contraction in response to changes in temperature can by itself aid in propagation of fractures in bedrock ranging in scale from intergranular fractures to widespread joints. Such a process is most effective in arid regions where the day-to-night temperature differences are great. It’s unclear whether the process is effective in thoroughly dry rocks, or whether the presence of water is an important element in the process. Experiments on heating and cooling of rock samples, over a great many cycles to simulate many decades or even centuries of the natural environment, have not been successful in producing non-negligible fracturing, but maybe the conditions of the simulation have not been representative. It’s well known, however, that fire causes breakdown of rock by sudden expansion of the surface layer exposed to the extreme heating.
frost wedging: Frost wedging (Figure 2-7) is important in climates where surface water is abundant and the day-to-night temperature range spans the freezing point many times through the year, as in New England in the late fall and especially the early spring. Water expands upon freezing, to the tune of about 9% in volume, an extremely large change. When water confined deeply in a thin crack in bedrock freezes, it can exert enormous side forces on the walls of the crack. The maximum possible force, which has been computed to be over 2000 kilograms per square centimeter (!), is not anywhere close to being achieved, however, because the ice does not seal the crack as strongly as the rock walls themselves, but the process is nonetheless very effective. Mountaintops with expanses of bedrock subjected to freeze–thaw cycles tend to be a jumble of frost-shattered rock; hikers in the Presidential Range in New Hampshire know that well.
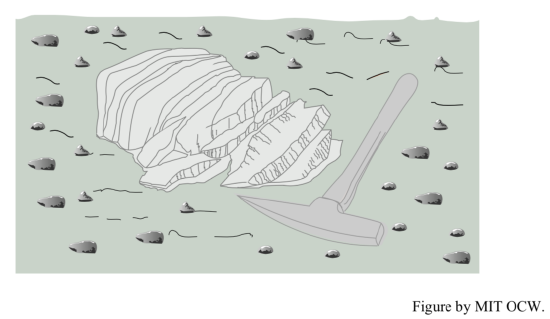
hydrofracturing: It’s thought by some investigators that the frost-wedging effect is more complicated than just simple expansion upon freezing. Water as thin films in very small cracks may stay liquid even at very low temperatures, for subtle thermodynamic reasons. As the freezing front progresses inward from the rock surface, water can be forced under high pressures into microscopic cracks, causing propagation of fractures. The phenomenon is known as hydrofracturing.
“boulder lifting”: It’s often said that boulders “grow” in New England fields. Frost action provides a good explanation for the effect (Figure 2-8). As the water-saturated ground freezes from the top down, freezing reaches the base of a large boulder sooner than it reaches the surrounding soil, because the rock of the boulder has a much lower heat capacity than the water-rich soil around it and is therefore cooled faster. Freezing of the water beneath the boulder lifts or pushes the boulder upward a short distance. Upon thawing of the ice beneath the boulder, the boulder does not fall as far as it was raised, because of the tendency for collapse and flowage of soil and finer rock fragments into the space beneath the boulder. Given enough cycles, the boulder makes its appearance at the surface.

root growth: Root growth can be very effective at widening preexisting cracks in bedrock near the surface. All of you have seen plants growing heroically in cracks in rock. In many cases the plant just occupies an already large crack, but in other cases it’s clear that the growing root has opened the crack.
Which of these various processes are the more important, and which are the less important? I can do no more than make an educated guess. (It depends, of course, on the particular setting of bedrock and climate, in any case.) In the right circumstances, frost wedging is clearly of great importance, but granular disintegration, and perhaps exfoliation, are probably of more general importance. Thermal expansion and contraction alone is generally considered to be less important than the other processes, except perhaps in particularly favored environments.
2.5.4 Weathering Profiles and Weathering Fronts
You’ve probably had the experience of picking up a broken stone and noticing that the outer part is colored reddish or brownish while the inner part looks a fresh gray. What’s going on is that the stone, having been derived from some outcrop as a fresh piece and then perhaps rounded at least somewhat by transport, rested somewhere at the Earth’s surface for a time long enough for the outer zone to have experienced some chemical weathering. The boundary between the outer, weathered zone and the inner, non-weathered zone is called the weathering front. There’s commonly a very abrupt change in the degree of weathering at the weathering front. In many cases the characteristic difference in color arises from the conversion of ferrous iron in minerals of the fresh rock to ferric iron oxides in the weathered zone.
The same effect tends to be present on a much larger scale when a bedrock landscape in an area of low relief is subjected to protracted weathering and the weathering products remain where they were formed. To see the effect, you need to have a vertical or steeply dipping cut down through the weathered rock, all the way down into fresh bedrock, which may be many meters or even tens of meters below the surface. Above the weathering front is a gradation from the most weathered material, at and near the land surface, down to the least weathered rock, at the weathering front. The succession of zones of degrees of weathering, from the surface down to the weathering front, is called a weathering profile.
Weathering profiles vary greatly in their nature, depending upon the factors listed in an earlier section (largely a matter of rock composition and climate). Figure 2-9 shows a common weathering profile, of the kind that tends to develop on deeply in-place-weathered coarse-grained igneous rocks like granite. The material just above the weathering front, called saprock, consists of partly chemically weathered minerals together with yet-unweathered minerals. The saprock preserves all of the original textures and structures of the parent rock, but its composition has been somewhat changed and its strength has been reduced. The saprock grades upward into material called saprolite, which is more altered chemically than the saprock below but still retains much of the textures and structures of the parent rock. You might describe such rock as “rotten rock” (and that is in fact the etymology of the term saprolite). Above the saprolite is even more strongly altered material, which has lost even more of the coherence of the parent and shows little of the original textures and structures. Above this, where the material has lost its coherence entirely and could be described as “loosed”, it is called regolith (see later section). The uppermost layer is the soil (see later section).
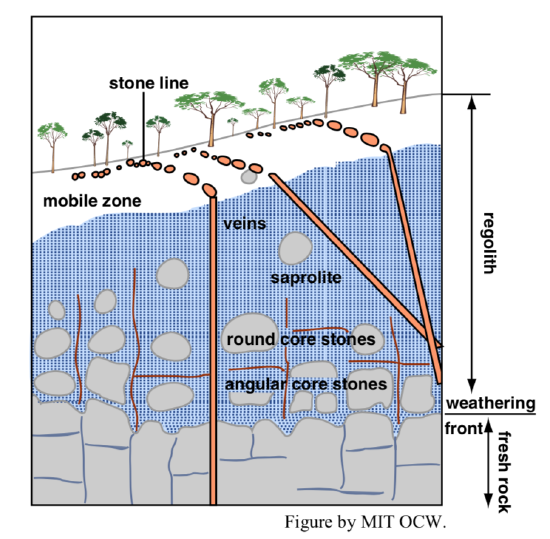
Note in Figure 2-9 that the geometry of the weathering front is strongly controlled by the presence of joints in the fresh rock. The weathering can make better progress along the joints than in the non-jointed intervening rock. In the process, there’s a tendency for non-weathered masses to be left behind as the weathering front progresses downward. These rounded, irregular masses are called corestones. They are similar to the weathering spheroids mentioned earlier, but they differ in being formed well below the land surface, whereas weathering spheroids develop at the rock surface as weathering proceeds at the same time that the weathering products are removed from the outcrop.