7.1: Introduction
- Page ID
- 15093
\( \newcommand{\vecs}[1]{\overset { \scriptstyle \rightharpoonup} {\mathbf{#1}} } \)
\( \newcommand{\vecd}[1]{\overset{-\!-\!\rightharpoonup}{\vphantom{a}\smash {#1}}} \)
\( \newcommand{\id}{\mathrm{id}}\) \( \newcommand{\Span}{\mathrm{span}}\)
( \newcommand{\kernel}{\mathrm{null}\,}\) \( \newcommand{\range}{\mathrm{range}\,}\)
\( \newcommand{\RealPart}{\mathrm{Re}}\) \( \newcommand{\ImaginaryPart}{\mathrm{Im}}\)
\( \newcommand{\Argument}{\mathrm{Arg}}\) \( \newcommand{\norm}[1]{\| #1 \|}\)
\( \newcommand{\inner}[2]{\langle #1, #2 \rangle}\)
\( \newcommand{\Span}{\mathrm{span}}\)
\( \newcommand{\id}{\mathrm{id}}\)
\( \newcommand{\Span}{\mathrm{span}}\)
\( \newcommand{\kernel}{\mathrm{null}\,}\)
\( \newcommand{\range}{\mathrm{range}\,}\)
\( \newcommand{\RealPart}{\mathrm{Re}}\)
\( \newcommand{\ImaginaryPart}{\mathrm{Im}}\)
\( \newcommand{\Argument}{\mathrm{Arg}}\)
\( \newcommand{\norm}[1]{\| #1 \|}\)
\( \newcommand{\inner}[2]{\langle #1, #2 \rangle}\)
\( \newcommand{\Span}{\mathrm{span}}\) \( \newcommand{\AA}{\unicode[.8,0]{x212B}}\)
\( \newcommand{\vectorA}[1]{\vec{#1}} % arrow\)
\( \newcommand{\vectorAt}[1]{\vec{\text{#1}}} % arrow\)
\( \newcommand{\vectorB}[1]{\overset { \scriptstyle \rightharpoonup} {\mathbf{#1}} } \)
\( \newcommand{\vectorC}[1]{\textbf{#1}} \)
\( \newcommand{\vectorD}[1]{\overrightarrow{#1}} \)
\( \newcommand{\vectorDt}[1]{\overrightarrow{\text{#1}}} \)
\( \newcommand{\vectE}[1]{\overset{-\!-\!\rightharpoonup}{\vphantom{a}\smash{\mathbf {#1}}}} \)
\( \newcommand{\vecs}[1]{\overset { \scriptstyle \rightharpoonup} {\mathbf{#1}} } \)
\( \newcommand{\vecd}[1]{\overset{-\!-\!\rightharpoonup}{\vphantom{a}\smash {#1}}} \)
\(\newcommand{\avec}{\mathbf a}\) \(\newcommand{\bvec}{\mathbf b}\) \(\newcommand{\cvec}{\mathbf c}\) \(\newcommand{\dvec}{\mathbf d}\) \(\newcommand{\dtil}{\widetilde{\mathbf d}}\) \(\newcommand{\evec}{\mathbf e}\) \(\newcommand{\fvec}{\mathbf f}\) \(\newcommand{\nvec}{\mathbf n}\) \(\newcommand{\pvec}{\mathbf p}\) \(\newcommand{\qvec}{\mathbf q}\) \(\newcommand{\svec}{\mathbf s}\) \(\newcommand{\tvec}{\mathbf t}\) \(\newcommand{\uvec}{\mathbf u}\) \(\newcommand{\vvec}{\mathbf v}\) \(\newcommand{\wvec}{\mathbf w}\) \(\newcommand{\xvec}{\mathbf x}\) \(\newcommand{\yvec}{\mathbf y}\) \(\newcommand{\zvec}{\mathbf z}\) \(\newcommand{\rvec}{\mathbf r}\) \(\newcommand{\mvec}{\mathbf m}\) \(\newcommand{\zerovec}{\mathbf 0}\) \(\newcommand{\onevec}{\mathbf 1}\) \(\newcommand{\real}{\mathbb R}\) \(\newcommand{\twovec}[2]{\left[\begin{array}{r}#1 \\ #2 \end{array}\right]}\) \(\newcommand{\ctwovec}[2]{\left[\begin{array}{c}#1 \\ #2 \end{array}\right]}\) \(\newcommand{\threevec}[3]{\left[\begin{array}{r}#1 \\ #2 \\ #3 \end{array}\right]}\) \(\newcommand{\cthreevec}[3]{\left[\begin{array}{c}#1 \\ #2 \\ #3 \end{array}\right]}\) \(\newcommand{\fourvec}[4]{\left[\begin{array}{r}#1 \\ #2 \\ #3 \\ #4 \end{array}\right]}\) \(\newcommand{\cfourvec}[4]{\left[\begin{array}{c}#1 \\ #2 \\ #3 \\ #4 \end{array}\right]}\) \(\newcommand{\fivevec}[5]{\left[\begin{array}{r}#1 \\ #2 \\ #3 \\ #4 \\ #5 \\ \end{array}\right]}\) \(\newcommand{\cfivevec}[5]{\left[\begin{array}{c}#1 \\ #2 \\ #3 \\ #4 \\ #5 \\ \end{array}\right]}\) \(\newcommand{\mattwo}[4]{\left[\begin{array}{rr}#1 \amp #2 \\ #3 \amp #4 \\ \end{array}\right]}\) \(\newcommand{\laspan}[1]{\text{Span}\{#1\}}\) \(\newcommand{\bcal}{\cal B}\) \(\newcommand{\ccal}{\cal C}\) \(\newcommand{\scal}{\cal S}\) \(\newcommand{\wcal}{\cal W}\) \(\newcommand{\ecal}{\cal E}\) \(\newcommand{\coords}[2]{\left\{#1\right\}_{#2}}\) \(\newcommand{\gray}[1]{\color{gray}{#1}}\) \(\newcommand{\lgray}[1]{\color{lightgray}{#1}}\) \(\newcommand{\rank}{\operatorname{rank}}\) \(\newcommand{\row}{\text{Row}}\) \(\newcommand{\col}{\text{Col}}\) \(\renewcommand{\row}{\text{Row}}\) \(\newcommand{\nul}{\text{Nul}}\) \(\newcommand{\var}{\text{Var}}\) \(\newcommand{\corr}{\text{corr}}\) \(\newcommand{\len}[1]{\left|#1\right|}\) \(\newcommand{\bbar}{\overline{\bvec}}\) \(\newcommand{\bhat}{\widehat{\bvec}}\) \(\newcommand{\bperp}{\bvec^\perp}\) \(\newcommand{\xhat}{\widehat{\xvec}}\) \(\newcommand{\vhat}{\widehat{\vvec}}\) \(\newcommand{\uhat}{\widehat{\uvec}}\) \(\newcommand{\what}{\widehat{\wvec}}\) \(\newcommand{\Sighat}{\widehat{\Sigma}}\) \(\newcommand{\lt}{<}\) \(\newcommand{\gt}{>}\) \(\newcommand{\amp}{&}\) \(\definecolor{fillinmathshade}{gray}{0.9}\)Soil Chemistry is the branch of soil science that deals with the chemical composition, chemical properties, and chemical reactions in soil. The soil environment is dynamic and comprised of a heterogeneous mixture of air, water, and inorganic and organic solids. Soil chemistry is primarily concerned with the chemical reactions associated with the many phases incorporated within the soil mixture. Soil chemistry is an ever-expanding field that traditionally focused on chemical reactions that affected plant growth and nutrition, and has since expanded to include water and soil contaminants and their effects on plants, animals, and humans.
Soil is made up of inorganic and organic assemblages, in the form of solids, liquids, and gases. Elemental content varies among soil types, however, the elements found in the highest quantities are typically O, Si, Al, Fe, C, Ca, K, Na, and Mg (in order of highest to lowest natural abundance). Inorganic components of soil makeup approximately 90% of all solid components, however, soil organic matter (SOM) still plays a critical role in chemical reactions within the soil. In addition, properties such as size, surface area, and charge behavior affect the majority of the essential equilibrium and kinetic reactions and processes which occur in soils (Figure 1).
In this lab we will cover important chemical reactions in soil, the properties that affect chemical reactions in the soil, and how to measure specific chemical properties in soils.
SOIL COLLOIDS
The extremely small, colloidal particles (smaller than 0.002 mm) of clay and humus control many important chemical and physical properties of the soil. This portion of the soil is often called the "active fraction", comprised of highly reactive materials with electrically charged surfaces. The small size of colloids results in a large surface area per unit weight, and their ionic structure results in a net electrical charge.
The type, amount, and mineralogy of colloids will strongly influence most land management decisions. For example a soil that is 40% of clay that primarily consists of smectite (a 2:1 shrink-swell clay) could have limitations for constructing roads, or building foundations due to the shifting of the soil as the soil wets and dries. Such a soil could be highly productive for row crop agriculture though, due to the high amount of charge that facilitates the retention of nutrients like Ca2+, K+, Mg2+, etc. On the contrary, a soil such as an Oxisol that has 80% clay has colloids that are primarily aluminum and iron oxides, which do not shrink or swell, and have a low amount of charge. Thus, the soil would be well suited for building foundations.
General properties of soil colloids
Size: smaller than what can be seen with a normal light microscope
Surface area: The smaller the size of the soil particles the greater the surface area. Higher surface area increases the availability of reaction sites for processes such as adsorption, catalysis, precipitation, microbial colonization, etc. Some colloids may also possess extensive internal surfaces, depending on crystalline units present.
Surface charge: colloids carry positive and/or negative electrostatic charges, whereby negative charges typically outweigh positive charges. Charge is sometimes pH dependent. Colloid charge helps to attract or repulse various substances in the soil solution or environment.
Types of soil colloids
Crystalline silicate clays: Clay types vary based on the number and combinations of structural units. The structural units are characterized by either tetrahedral units (Si4+) or octahedral units (Al3+, Mg2+, and Fe2+/Fe3+) (Figure 2). Individual units link together to form “sheets” of tightly bound and tightly packed 0-Si-Al atoms, which combine to form the layers which give the clay its characteristic crystalline structure. The size and location of layer charge varies due to the process of isomorphic substitution, which occurs in the layered sheets (Figure 2). Crystalline silicate Clays are typically categorized into two categories: 1) 1:1 clays; and, 2) 2:1 clays.
1:1 Clays- (i.e. Kaolinite)
- One tetrahedron and one octahedron sheet
- Non-expanding, no shrink-swell action
- Little isomorphic substitution
- Found in highly weathered soils
2:1 Clays- (i.e. smectite, vermiculite, and mica)
- One octahedral sheet between two tetrahedral sheets
- Have a variety of shrink-swell potentials, favoring increased shrink-swell action
- Charge dominated by isomorphic substitution
- Isomorphic substitution occurs in the… octahedral (smectite), tetrahedral (mica), and tetrahedral (vermiculite)
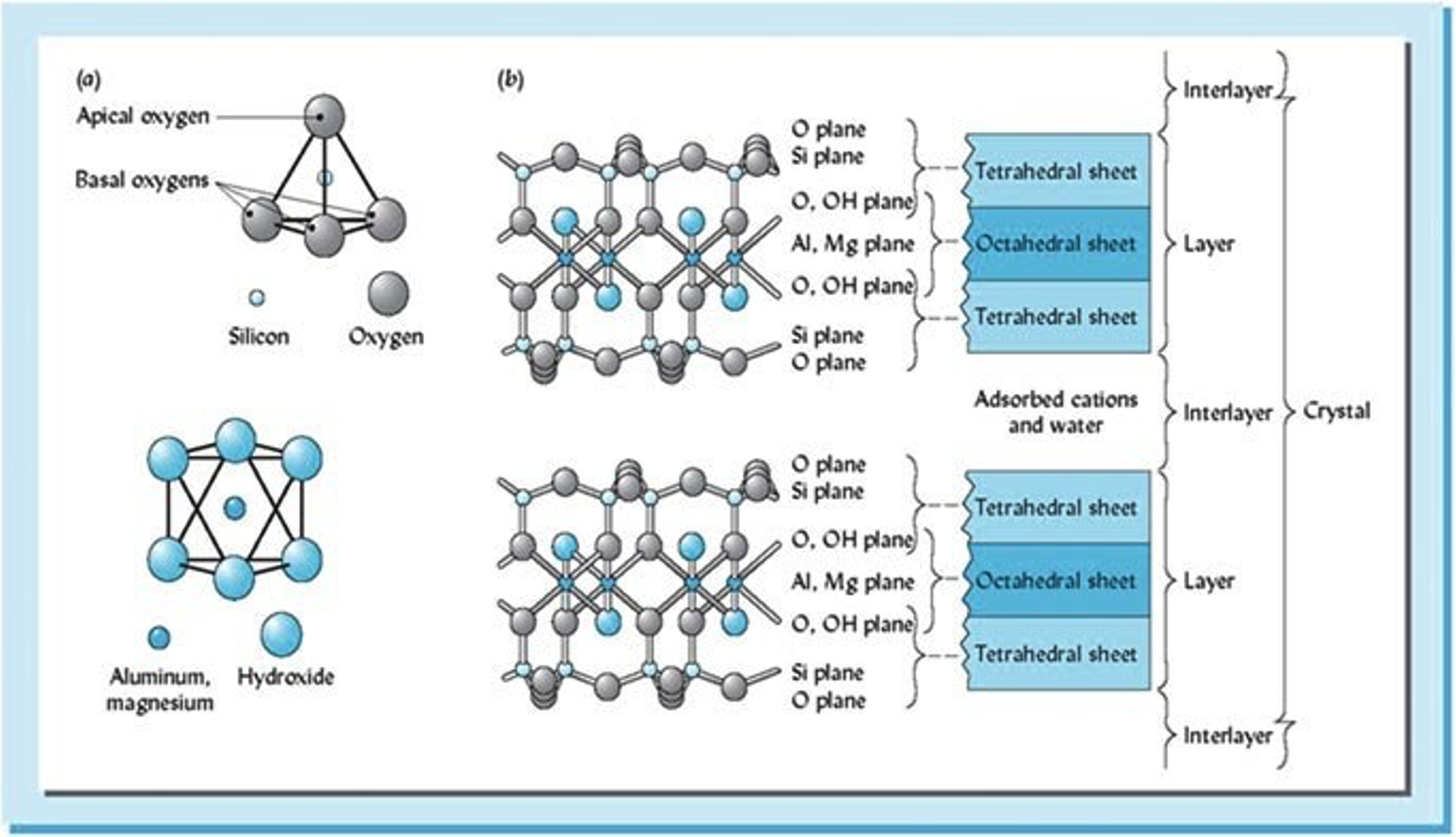
Noncrystalline silicate clays: These clays also consist of tightly bonded Si-Al-O atoms, oriented into unordered, noncrystalline sheets. They are characterized by high water holding capacity and extremely high capacities to strongly absorb phosphate and other anions, particularly under acid conditions. Examples of noncrystalline silicate clays include, allophane and imogolite, typically found in Andisols, or soils derived from volcanic ash.
Iron and aluminum oxides: these properties are present in many soils but are highly expressed in highly weathered soils of warm, humid regions. They consist of either Fe or Al atoms combined with O atoms, with a net charge that ranges from slightly negative to moderately positive. Examples of iron oxides include crystalline goethite and soil coatings. Examples of aluminum oxides include crystalline gibbsite and soil coatings.
Organic material/humus: Organic colloids or humus, are especially important in the upper portions of a soil profile. Because these colloids are not mineral material, alternatively, they consist of complex chains and rings of carbon atoms bonded to H, O, and N. These colloids are often the smallest in size, have a net negative charge that varies with soil pH, and have a high capacity to absorb water.
SOIL CHARGE
Now that we have prefaced the basics of soil colloids, it is important to highlight some of the important processes which contribute to the potential charge of a soil. To begin, there are two kinds of charge within a soil: 1) Permanent charge and 2) Variable charge.
- Permanent charge: this charge is determined from isomorphic substitution which takes place in the clay fraction of the soil between phyllosilicate layers of crystalline silicate clays.
- Variable charge: this charge is predominantly pH dependent and results from reaction of OH- associated with the following
- Edges of clay minerals
- Organic material
- Aluminum and iron oxides
Isomorphic substitution
Is the process by which one element fills a position originally filled by another element of similar size (ionic radii). This process typically occurs between Si, Al, and Mg ions held within octahedral and tetrahedral structures, whereby they are replaced by a cation of similar size (Figure 3). This replacement alters the overall charge and nature of silicate clays. For example, if Mg2+ substitutes Al3+, overall charge balances in favor of a negative charge from the presence of O, and the resulting sheet is left with a net negative charge (Figure 4).
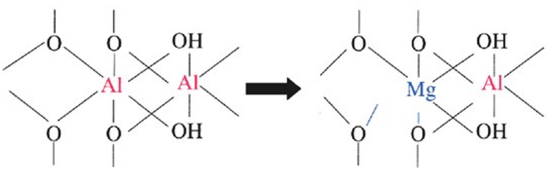
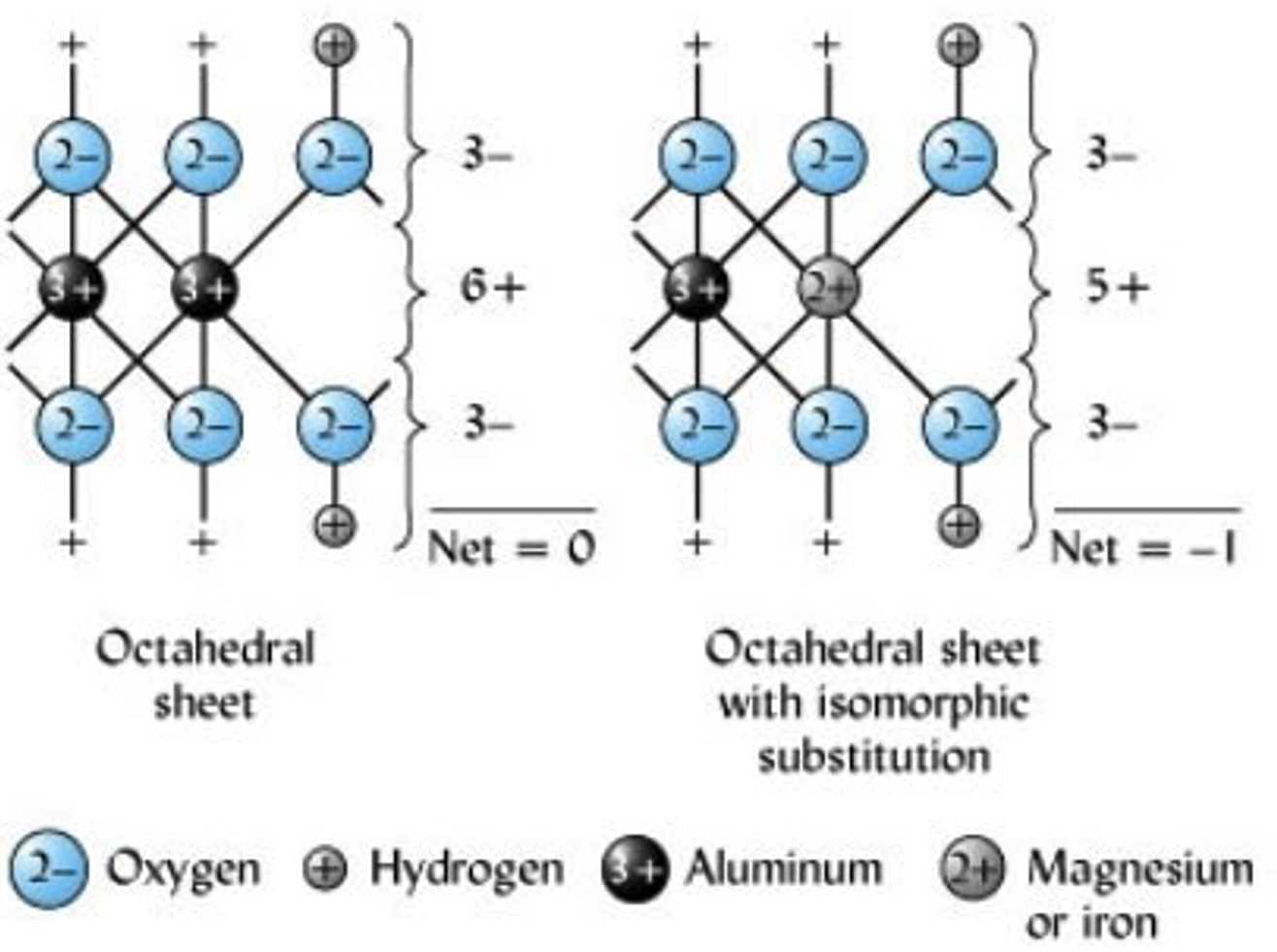
Cation Exchange Capacity
Ion exchange is one of the most significant features of the clay and humus fractions. The capacity of the particles to attract or adsorb cations is called the cation exchange capacity (CEC). This soil property is very important for plant uptake of nutrients and buffering against soil acidification. Clay minerals and organic components of the soil have negatively charged sites which can absorb positively charged cations and hold those cations on their surfaces by electrostatic force. This ability allows the soil to serve as a storehouse of plant nutrients like potassium (K+), calcium (Ca2+), and magnesium (Mg2+), also referred to as base cations. Measuring base cations in a soil will give a good idea of that soil’s CEC.
Because many plant nutrients exist as cations, CEC is critical for plant growth. A soil with high clay and organic matter will have more negatively charged sites for cations and therefore have a greater CEC and are more fertile for plants. However, in an agricultural setting, crops can be grown on soils with low CEC and still be productive with amendments. The reactive exchange capacity of the soil also permits it to serve as a filter or treatment medium for land application of waste materials.
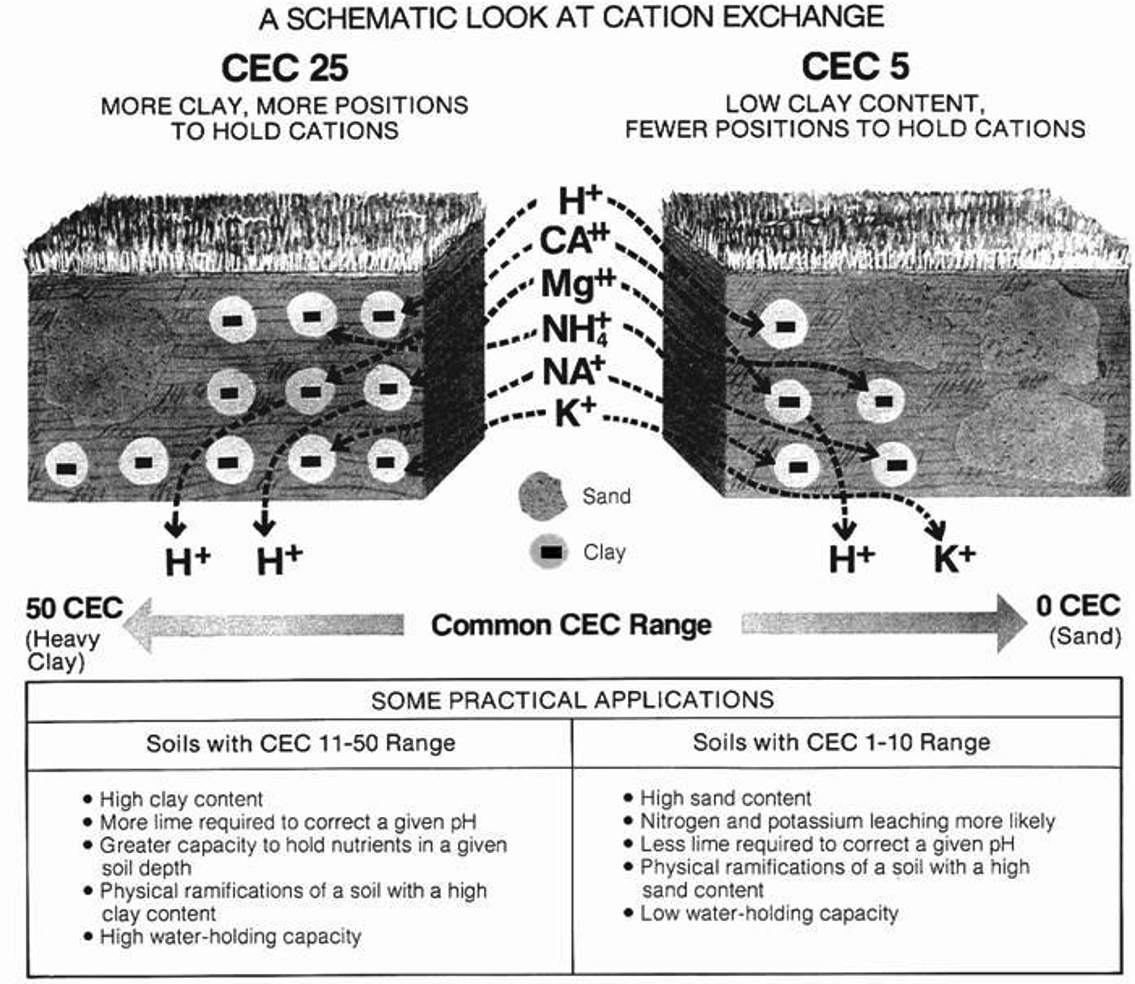
Determining Cation Exchange Capacity
The cation exchange capacity (quantity of cations a soil can adsorb per unit weight, CEC) can be determined using a simple displacement process (Figure 5).
- Soil sample is first saturated with a simple cation like NH4 + so all the negative charge sites are occupied by NH4+.
- Excess NH4+ (i.e., not on exchange sites) is removed by leaching with ethyl alcohol.
- Another cation such as Ba2+ is used to displace all the NH4+. The NH4 + is collected in the filtrate and measured. The quantity of NH4+ collected from the sample is the quantity of cations that the soil can hold, i.e. CEC.
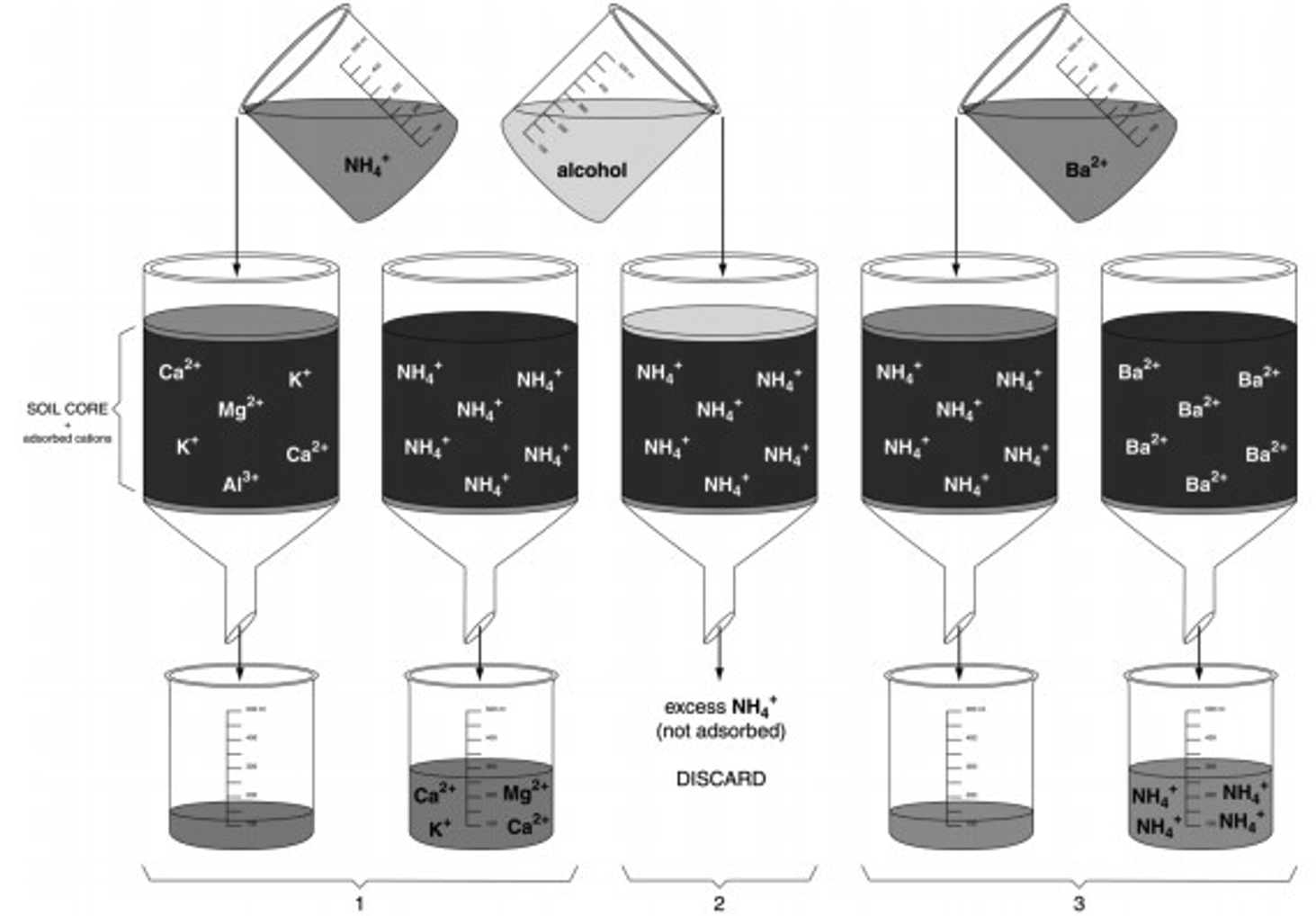
Calculating CEC
The Sum-of-Cations Method: If you have a soil analysis where the quantities of all cations in the soil are listed, simply summing all those exchangeable quantities will yield the CEC you found in the preceding problems.
The "Mineralogy" Method: As you know from your reading and class discussion, clay minerals have a range of values for CEC. If the mineralogy of the clay fraction is known (that is, the type and amounts of each clay mineral), then the CEC can be approximated.
To make these calculations easier, Table 1 contains representative values for CEC to use in all calculations for this class unless otherwise noted. In nature, however, these soil colloids will have a range of values.
Mineral or colloid type | CEC of pure colloid (cmolc/kg) |
---|---|
kaolinite | 10 |
illite | 30 |
montmorillonite/smectite | 100 |
vermiculite | 150 |
humus | 200 |
Example: Using the mineralogy approach to CEC calculations, consider a soil having 100% clay where the clay is 100% kaolinite. The CEC would then be 10 cmolc/kg. If a soil contains only 10% kaolinite (or 10 kg clay in 100 kg soil), however, this clay would contribute:
\[ \mathrm{Total\; CEC\; of\; the\; soil = \dfrac{10 \;cmol_c}{kg\;clay} \times \dfrac{10 \;kg \;clay}{100 \;kg\;soil} = \dfrac{1.0 \;cmol_c}{kg\;soil}} \nonumber \]
Soil pH
By definition, pH = -log [H+]. The pH of soil is a critical factor for the fate of many chemical processes and the variable charge of soil. Soil pH is also incredibly important for plant growth: certain plant essential nutrients are only available within certain pH ranges. Plant optimal pH ranges vary greatly. Some plants excel in low pHs (e.g. blueberries) while others are adapted to high pHs (e.g. asparagus) (Figure 7). For agriculture, if the soil pH is not desirable for the given crop, it can be adjusted. The addition of lime can raise the pH while adding organic matter or ammonium fertilizer can lower the pH.
Soil Electrical Conductivity
Electrical Conductivity (EC) is the measure of the amount of salts in soil, or salinity. EC varies by soil type, texture, and location (Figure 8). EC is very relevant in the Western U.S. as well as in tidal zones in coastal areas. EC affects multiple facets of soil health including soil-water balance and soil microbe activity. The negative effects on the soil-water balance can be devastating to plants: saline soils have a lower water potential than what is within plants, causing water to move from the plants to the soil. This loss of water causes drought stress to the plants. High levels of salinity are common in the West because of the drier climate. With lack of water movement through the soil profile the salts are not flushed out of the soil and accumulate near the surface. There are many ways to properly manage a soil that has an EC higher than desired, including limiting nitrogen fertilizer (which can increase salinity) as well as practicing no-till agriculture to retain soil moisture.
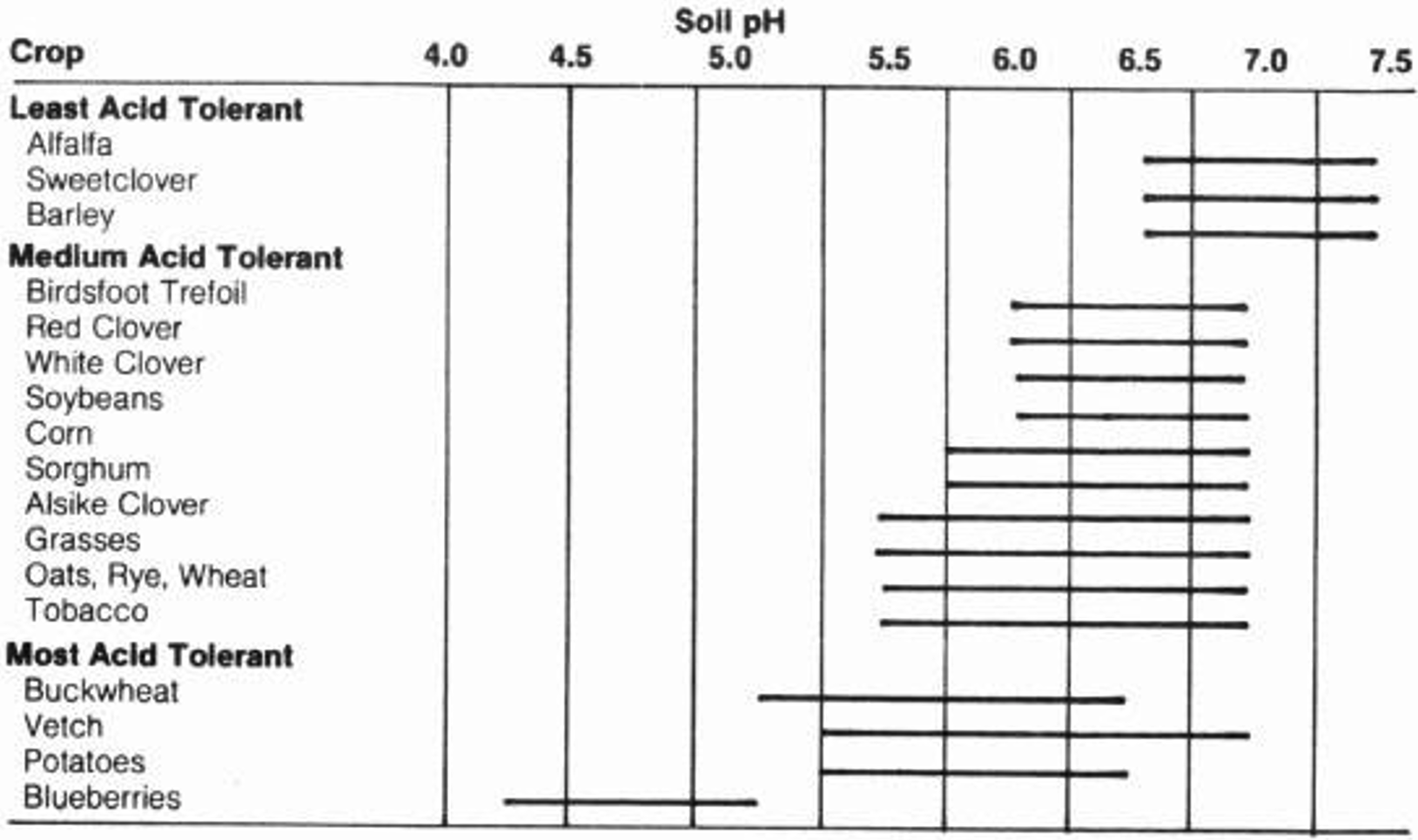
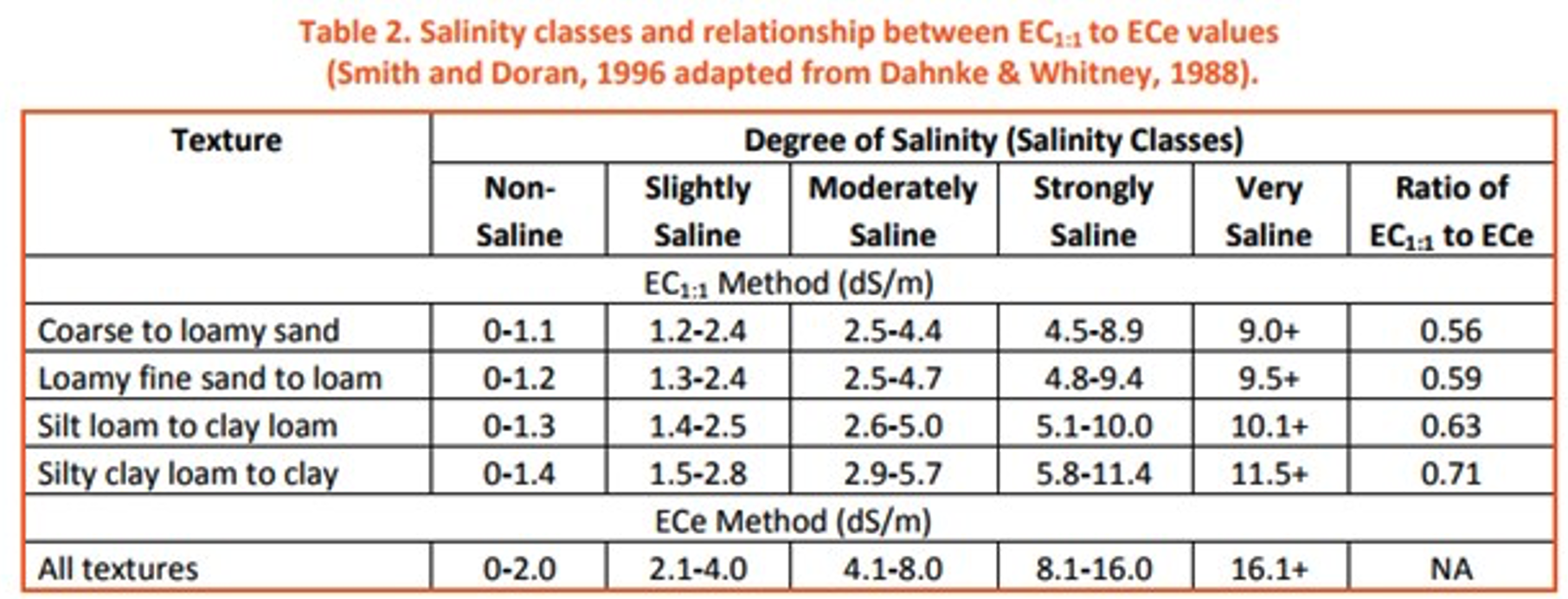
REDUCTION-OXIDATION (redox) REACTIONS IN SOIL
Alternating oxidizing and reducing conditions in soils influence a number of critical chemical reactions, the potential for plant growth, soil organic matter dynamics, and the survival of organisms. The quantification of reducing soil conditions is driven by saturated soil conditions and is particularly important in the assessment of wetland function and identification of hydric soils.
The redox ladder (figure 8) serves as a useful guide indicating the hierarchy of chemical compounds used as terminal electron acceptors (TEA) during microbially mediated reduction reactions (Figure 9). The various compounds are utilized as TEAs at specific redox potential (Eh) ranges and are impacted by pH as well.
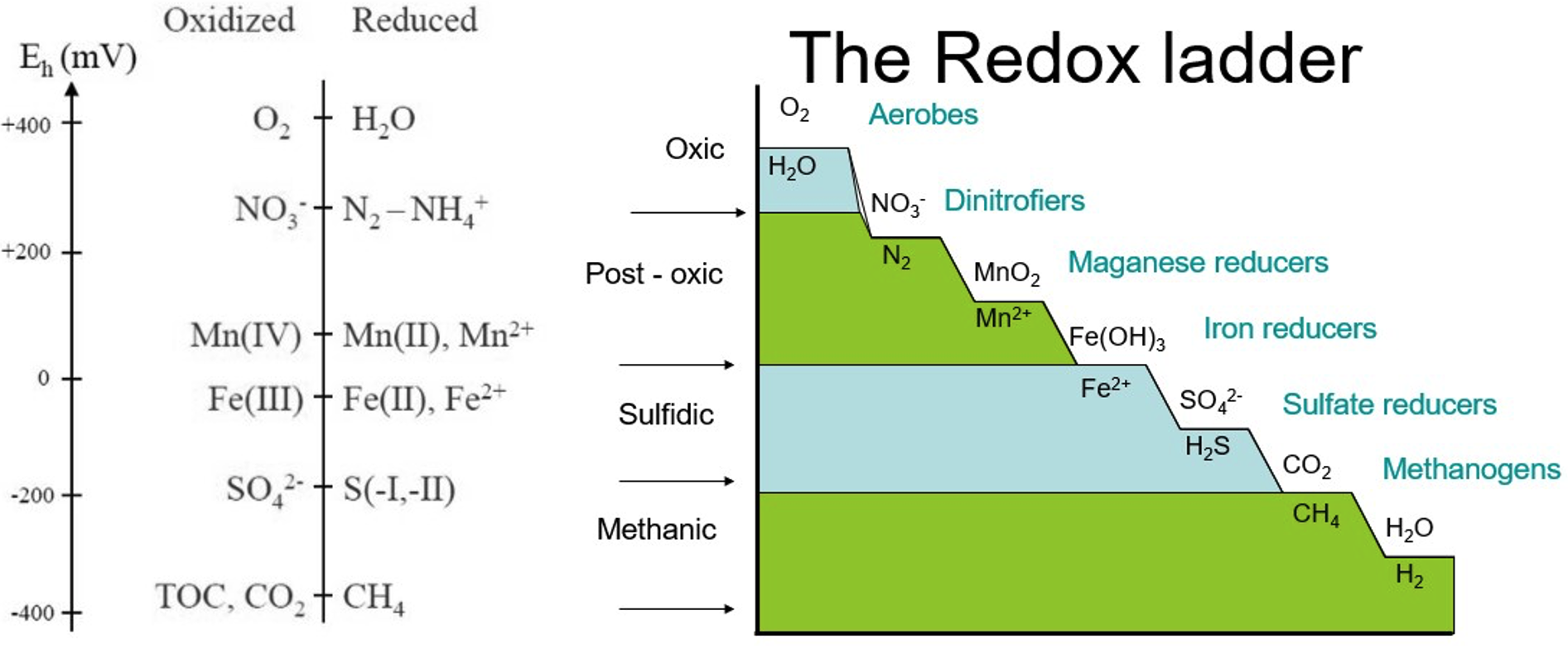
Redox Diagrams
Redox conditions in soil are represented on a Redox diagram, which displays Eh versus pH (Figure 10). By determining the pH and the Eh and finding the point of intersection on the Redox diagram one can obtain a fairly good idea about the general nature of the soil system.
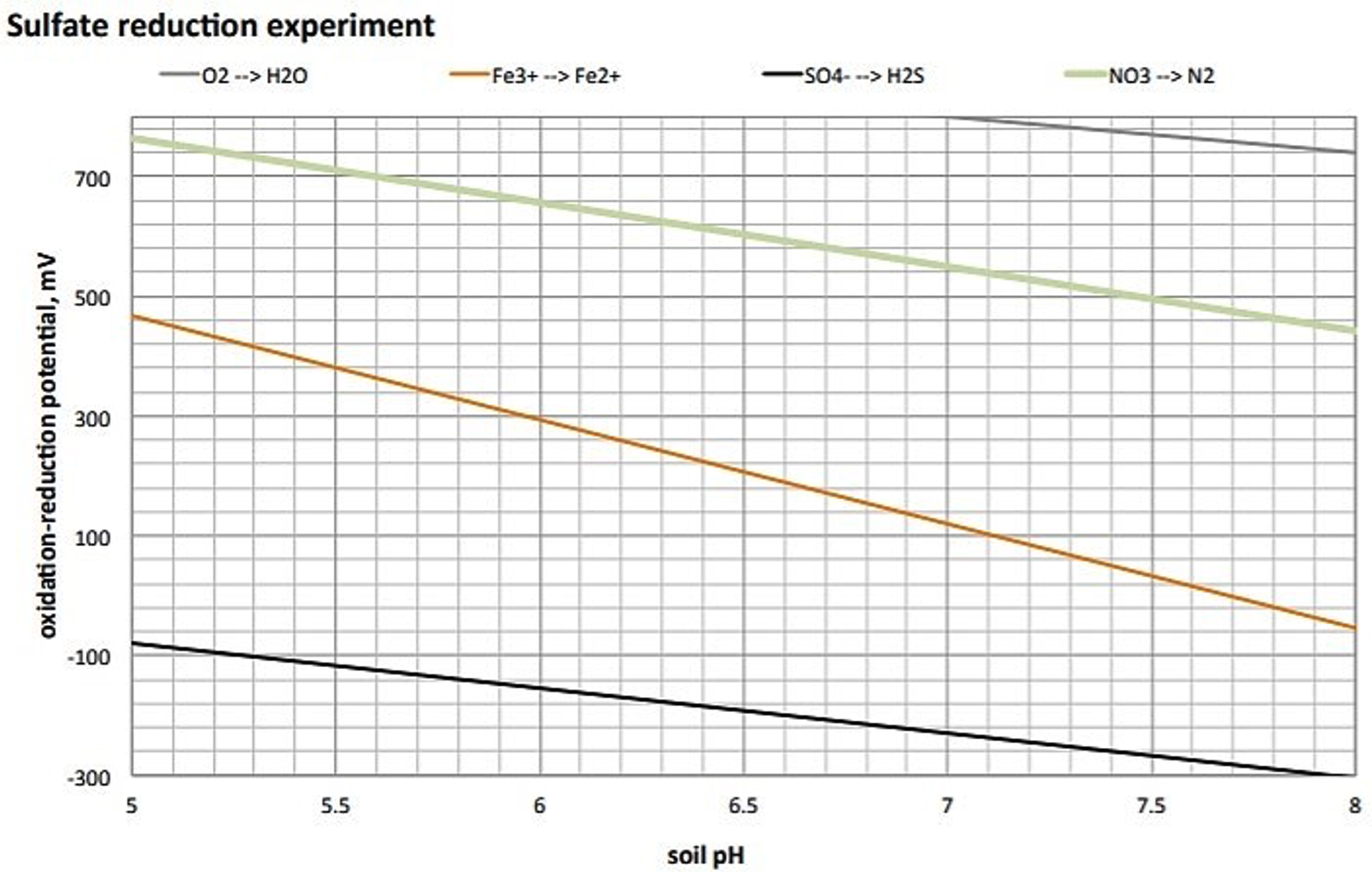
Effects of reducing soil conditions on the soil environment
Anaerobic conditions can generate methane gas. The gas may be visible as entrapped bubbles in the suspension. Also, the anaerobic conditions can be appreciated by carefully detecting the nature of the system using your olfactory senses (smell). Under anaerobic conditions, microorganisms are prone to generate many organic amines (such as putrescine), which are highly odoriferous. Hydrogen sulfide (rotten egg) gas can be generated when the system becomes strongly anaerobic and most of the metal ions have been converted to FeS or FeS2.
Sulfate reduction occurs under anaerobic conditions where the sulfate ion serves as an alternate electron acceptor replacing the normal oxygen gas molecule. Energy must be provided to the microorganisms in the form of readily available organic material, which provides the electrons in the H atoms that are never isolated as such in the reaction. The reaction may be summarized as follows:
\[ SO_4^2 + 8[H^+] \rightarrow S^{2-} + 4H_2O \nonumber \]
The sulfide that forms is very reactive. It forms extremely insoluble metal sulfides (iron-monosulfides (FeS) and pyrites (FeS2)). These metal sulfides give the solution a black or dark gray appearance. Often an organic mat forms on the surface of these soils.
Please read through and complete the following activity and questions. All questions should be answered and completed labs are due at the end of the laboratory period. No late work will be accepted.