13.1: Front Matter
- Page ID
- 14570
\( \newcommand{\vecs}[1]{\overset { \scriptstyle \rightharpoonup} {\mathbf{#1}} } \)
\( \newcommand{\vecd}[1]{\overset{-\!-\!\rightharpoonup}{\vphantom{a}\smash {#1}}} \)
\( \newcommand{\id}{\mathrm{id}}\) \( \newcommand{\Span}{\mathrm{span}}\)
( \newcommand{\kernel}{\mathrm{null}\,}\) \( \newcommand{\range}{\mathrm{range}\,}\)
\( \newcommand{\RealPart}{\mathrm{Re}}\) \( \newcommand{\ImaginaryPart}{\mathrm{Im}}\)
\( \newcommand{\Argument}{\mathrm{Arg}}\) \( \newcommand{\norm}[1]{\| #1 \|}\)
\( \newcommand{\inner}[2]{\langle #1, #2 \rangle}\)
\( \newcommand{\Span}{\mathrm{span}}\)
\( \newcommand{\id}{\mathrm{id}}\)
\( \newcommand{\Span}{\mathrm{span}}\)
\( \newcommand{\kernel}{\mathrm{null}\,}\)
\( \newcommand{\range}{\mathrm{range}\,}\)
\( \newcommand{\RealPart}{\mathrm{Re}}\)
\( \newcommand{\ImaginaryPart}{\mathrm{Im}}\)
\( \newcommand{\Argument}{\mathrm{Arg}}\)
\( \newcommand{\norm}[1]{\| #1 \|}\)
\( \newcommand{\inner}[2]{\langle #1, #2 \rangle}\)
\( \newcommand{\Span}{\mathrm{span}}\) \( \newcommand{\AA}{\unicode[.8,0]{x212B}}\)
\( \newcommand{\vectorA}[1]{\vec{#1}} % arrow\)
\( \newcommand{\vectorAt}[1]{\vec{\text{#1}}} % arrow\)
\( \newcommand{\vectorB}[1]{\overset { \scriptstyle \rightharpoonup} {\mathbf{#1}} } \)
\( \newcommand{\vectorC}[1]{\textbf{#1}} \)
\( \newcommand{\vectorD}[1]{\overrightarrow{#1}} \)
\( \newcommand{\vectorDt}[1]{\overrightarrow{\text{#1}}} \)
\( \newcommand{\vectE}[1]{\overset{-\!-\!\rightharpoonup}{\vphantom{a}\smash{\mathbf {#1}}}} \)
\( \newcommand{\vecs}[1]{\overset { \scriptstyle \rightharpoonup} {\mathbf{#1}} } \)
\( \newcommand{\vecd}[1]{\overset{-\!-\!\rightharpoonup}{\vphantom{a}\smash {#1}}} \)
\(\newcommand{\avec}{\mathbf a}\) \(\newcommand{\bvec}{\mathbf b}\) \(\newcommand{\cvec}{\mathbf c}\) \(\newcommand{\dvec}{\mathbf d}\) \(\newcommand{\dtil}{\widetilde{\mathbf d}}\) \(\newcommand{\evec}{\mathbf e}\) \(\newcommand{\fvec}{\mathbf f}\) \(\newcommand{\nvec}{\mathbf n}\) \(\newcommand{\pvec}{\mathbf p}\) \(\newcommand{\qvec}{\mathbf q}\) \(\newcommand{\svec}{\mathbf s}\) \(\newcommand{\tvec}{\mathbf t}\) \(\newcommand{\uvec}{\mathbf u}\) \(\newcommand{\vvec}{\mathbf v}\) \(\newcommand{\wvec}{\mathbf w}\) \(\newcommand{\xvec}{\mathbf x}\) \(\newcommand{\yvec}{\mathbf y}\) \(\newcommand{\zvec}{\mathbf z}\) \(\newcommand{\rvec}{\mathbf r}\) \(\newcommand{\mvec}{\mathbf m}\) \(\newcommand{\zerovec}{\mathbf 0}\) \(\newcommand{\onevec}{\mathbf 1}\) \(\newcommand{\real}{\mathbb R}\) \(\newcommand{\twovec}[2]{\left[\begin{array}{r}#1 \\ #2 \end{array}\right]}\) \(\newcommand{\ctwovec}[2]{\left[\begin{array}{c}#1 \\ #2 \end{array}\right]}\) \(\newcommand{\threevec}[3]{\left[\begin{array}{r}#1 \\ #2 \\ #3 \end{array}\right]}\) \(\newcommand{\cthreevec}[3]{\left[\begin{array}{c}#1 \\ #2 \\ #3 \end{array}\right]}\) \(\newcommand{\fourvec}[4]{\left[\begin{array}{r}#1 \\ #2 \\ #3 \\ #4 \end{array}\right]}\) \(\newcommand{\cfourvec}[4]{\left[\begin{array}{c}#1 \\ #2 \\ #3 \\ #4 \end{array}\right]}\) \(\newcommand{\fivevec}[5]{\left[\begin{array}{r}#1 \\ #2 \\ #3 \\ #4 \\ #5 \\ \end{array}\right]}\) \(\newcommand{\cfivevec}[5]{\left[\begin{array}{c}#1 \\ #2 \\ #3 \\ #4 \\ #5 \\ \end{array}\right]}\) \(\newcommand{\mattwo}[4]{\left[\begin{array}{rr}#1 \amp #2 \\ #3 \amp #4 \\ \end{array}\right]}\) \(\newcommand{\laspan}[1]{\text{Span}\{#1\}}\) \(\newcommand{\bcal}{\cal B}\) \(\newcommand{\ccal}{\cal C}\) \(\newcommand{\scal}{\cal S}\) \(\newcommand{\wcal}{\cal W}\) \(\newcommand{\ecal}{\cal E}\) \(\newcommand{\coords}[2]{\left\{#1\right\}_{#2}}\) \(\newcommand{\gray}[1]{\color{gray}{#1}}\) \(\newcommand{\lgray}[1]{\color{lightgray}{#1}}\) \(\newcommand{\rank}{\operatorname{rank}}\) \(\newcommand{\row}{\text{Row}}\) \(\newcommand{\col}{\text{Col}}\) \(\renewcommand{\row}{\text{Row}}\) \(\newcommand{\nul}{\text{Nul}}\) \(\newcommand{\var}{\text{Var}}\) \(\newcommand{\corr}{\text{corr}}\) \(\newcommand{\len}[1]{\left|#1\right|}\) \(\newcommand{\bbar}{\overline{\bvec}}\) \(\newcommand{\bhat}{\widehat{\bvec}}\) \(\newcommand{\bperp}{\bvec^\perp}\) \(\newcommand{\xhat}{\widehat{\xvec}}\) \(\newcommand{\vhat}{\widehat{\vvec}}\) \(\newcommand{\uhat}{\widehat{\uvec}}\) \(\newcommand{\what}{\widehat{\wvec}}\) \(\newcommand{\Sighat}{\widehat{\Sigma}}\) \(\newcommand{\lt}{<}\) \(\newcommand{\gt}{>}\) \(\newcommand{\amp}{&}\) \(\definecolor{fillinmathshade}{gray}{0.9}\)What Is the Rock Cycle?
The rock cycle is a circular diagram that demonstrates the process by which rocks are continually recycled throughout geologic time. All rocks can be classified into one of three groups: igneous, sedimentary, or metamorphic. This rock classification is based on the origin of each of these rock types, or the processes that form the rock. The Rock Cycle (Figure 13.1), represents the processes that produce each of the rock types. In Chapter 7, we explored igneous rocks, which are produced through the melting of rock and eventual cooling of lava or magma; as cooling occurs, crystals may form. In Chapter 11, we investigated sedimentary rocks, which are produced via the processes of weathering (the chemical and physical breakdown of material at Earth’s surface that produces sediments), erosion (the movement and deposition of sediments), and lithification (the compaction and cementation of sediments). We’ve now reached metamorphic rocks, which are produced via the agents of change, primarily heat and pressure that typically result in the recrystallization of minerals.

What Is a Metamorphic Rock?
The word metamorphism means to change form; for rocks, this means a recrystallization of minerals (crystals) under subsolidus conditions (temperatures too low for melt production). All rocks form at certain temperatures and pressures at, or more commonly, beneath the Earth’s surface. These rocks are most stable at the conditions under which they form. Therefore, changing the temperature and/or pressure conditions may lead to a different rock, one that changed in order to be stable under the new external conditions. This new rock that forms in response to changes in its physical and chemical environment is called a metamorphic rock. A metamorphic change can also occur if the rock’s composition is altered by hot, chemically reactive fluids, causing a change in the mineral content of the rock.
Metamorphic rocks form by the physical and sometimes chemical alteration of a pre-existing rock. To distinguish between the pre-existing rock and the new metamorphic one, the term protolith (proto- meaning “original” and -lith meaning “rock”), or parent rock, is used to describe the pre-existing rock (Figure 13.2). All metamorphic rocks have at least one protolith that was altered during metamorphism. Igneous, sedimentary, or in some cases, even metamorphic rocks can be altered into a completely new or different metamorphic rock.
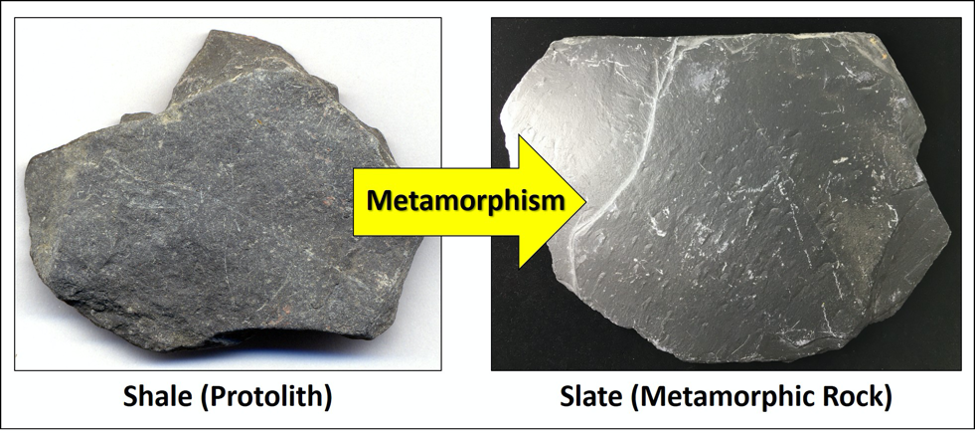
A person who studies metamorphic rocks is typically referred to as a metamorphic petrologist. A petrologist is a geoscientist that studies rocks and the conditions under which rocks form. A metamorphic petrologist specializes in metamorphic rocks and their unique conditions of formation. Like many other geoscientists, working with other disciplines is common, with a heavy influence from both math and technology. Many are employed by universities where they teach and/or do research, and at state and federal agencies, including geological surveys, like the California Geological Survey or United State Geological Survey (USGS). Additional career pathways are available in the private sector including mining and natural resource extraction. Many of these career options require a college degree and postgraduate work. If you are interested, talk to your geology instructor for advice. We recommend completing as many math and science courses as possible (chemistry is incredibly important for mineralogy). Also, visit National Parks, CA State Parks, museums, gem & mineral shows, or join a local rock and mineral club. Typically, natural history museums will have wonderful displays of rocks, including those from your local region. Here in California, there are a number of large collections, including the San Diego Natural History Museum, Natural History Museum of Los Angeles County, Santa Barbara Museum of Natural History, and Kimball Natural History Museum. Many colleges and universities also have their own collections/museums.
Agents of Metamorphism
Pressure
All rocks beneath the surface of the earth experience an increase in pressure due to the weight of the overlying sediment and rock layers. Therefore, with increasing depth there is a corresponding increase in pressure. Typically, this lithostatic (confining) pressure is equal in all directions and will not necessarily cause a rock to become metamorphic (Figure 13.3A). Have you ever dived deep into a pool, lake, or ocean? As you descend, your eardrums experience hydrostatic pressure; this is similar to what sediments and rocks experience under lithostatic pressure. Lithostatic pressure may alter the overall rock or sediment volume but will not cause a change in overall shape. For example, lithostatic pressure can cause clasts to become more closely packed or reduce pore space within a clastic sedimentary rock.

Figure 13.3: Confining pressure (left) and differential pressure (right).
What happens if the pressure on a rock is unequal, and the rocks become squeezed in one direction more than another direction? This is known as differential (directional) pressure, and can result in a significant change in the appearance of a rock (Figure 13.3B). Imagine deformation happening to all the grains in the sedimentary rock or to all the crystals in an igneous rock. The resulting rock will have minerals aligned in a certain direction, through bond breaking and recrystallization. The result is a rock with a metamorphic pattern called a foliation. Metamorphic foliations are the patterns seen in a rock that has experienced differential pressure. Foliations may be flat or have a wavy appearance possibly due to more than one direction of greatest pressure (Figure 13.4). Some rocks may also develop what is called a lineation, which can be formed by an elongation of minerals that form a linear feature through the rock.

Temperature
Metamorphism often involves both an increase in temperature and pressure changes; however, the broad classification for metamorphism into low-, medium-, and high-grades of metamorphic change exists mainly due to temperature conditions. Higher temperatures increase the vibrational energy between the bonds linking atoms in the mineral structure, making it easier for bonds to be broken and minerals to recrystallize into new crystal shapes; high temperatures can also sometimes result in the development of foliation and lineation outlined above, even without differential pressure conditions. When temperatures are increased, there can be a corresponding increase in mineral sizes as initially small minerals become fused into larger crystals. This fusing of numerous smaller mineral sizes into fewer, yet larger, is also an example of recrystallization (Figure 13.5).

Why do increasing temperatures lead to increased grain sizes? In general, a mineral grain or crystal is most stable when it has a low surface area to volume ratio, therefore large grains are more stable than small grains. Increasing the grain size results in a greater increase in volume as opposed to a smaller increase in the surface area. Why does stability matter? Rocks become unstable when their environment changes, and through a recrystallization process (metamorphism), they can return to a stable form once again. Figure 13.5 demonstrates the recrystallization process in response to elevated temperature. In this example, the original grains are smaller and rounded, but recrystallization resulted in larger grains that become interlocking; the pore spaces are gone, and instead larger crystals exist.

In addition to increased grain size with increased temperature, occasionally a new mineral will form during metamorphism. These new minerals form at certain temperatures and are called index minerals, which can be used to determine the temperature of metamorphism (Figure 13.6).
Chemically Reactive Fluids
Higher temperatures are sometimes associated with metamorphism due to chemically reactive fluids. The phrase “chemically reactive” refers to the dissolved ions in a fluid phase that may react with minerals in a rock. These ions may take the place of some of the atoms in the mineral’s structure, which can lead to a significant change in the chemical composition of a rock. Sometimes these fluids are quite hot, especially if they are fluids released from a nearby magma body that is crystallizing while cooling. Metamorphism due to such fluids is known as hydrothermal metamorphism. California’s state rock, serpentinite, is formed during this process!
What Are Metamorphic Grades?
Metamorphic grade refers to the general temperature and pressure conditions that prevailed during metamorphism. As the pressure and temperature increase, rocks undergo metamorphism at higher metamorphic grade (Figure 13.7).
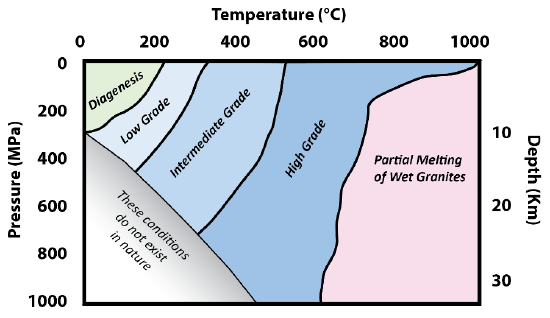
Very Low and Low-grade metamorphism takes place at approximately 150–300°C and relatively low pressure. This is not far beyond the conditions in which sediments are lithified into sedimentary rocks, and it is common for a low-grade metamorphic rock to look similar to its protolith. Low-grade metamorphic rocks tend to be characterized by an abundance of hydrous minerals, minerals that contain water within their crystal structure. Examples of low-grade hydrous minerals include clay, serpentine, and chlorite. Under low-grade metamorphism many of the metamorphic minerals will not grow large enough to be seen without a microscope.
Intermediate-grade metamorphism takes place at approximately 300–450°C and at moderate pressures. Low-grade hydrous minerals are replaced by micas such as biotite and muscovite, and non-hydrous minerals such as garnet may now grow. Garnet is an example of a mineral which may form porphyroblasts, metamorphic mineral grains that are larger in size and more equant in shape (about the same diameter in all directions), thus standing out among the smaller, flatter, or more elongate minerals.
High-grade metamorphism takes place at temperatures above about 450°C. Micas tend to break down. New minerals such as hornblende will form, which is stable at higher temperatures. As metamorphic grade increases to even higher grade, however, all hydrous minerals, including hornblende, may break down and be replaced by other, higher-temperature, non-hydrous minerals such as pyroxene.
How Do I Identify Metamorphic Rocks?
All metamorphic rocks are identified by their mineral content and texture. For metamorphic rocks, texture refers primarily to the orientation of the minerals in the rock. Additionally, crystal size can also convey important information regarding the temperature and pressure conditions during metamorphism.
To identify metamorphic rocks, you will first need to determine if the rock is foliated or non-foliated. Examine the rock for evidence of any pattern or foliation, and if present, identify the size and mineral(s) that compose the foliation pattern (Figure 13.8, left). Non-foliated metamorphic rocks can be identified by the properties associated with their dominant composition (Figure 13.8, right).

What Is a Foliated Metamorphic Rock?
Differential pressure typically results in the development of foliation in metamorphosed rocks. Metamorphic rocks have a variety of foliation examples; however, each is dependent on the minerals that define the foliation.
The most obvious is gneissic banding, which can be identified by the alternating dark and light mineral bands throughout the rock (Figure 13.9). The resulting metamorphic rock is called gneiss (pronounced “nice”, with a silent g). These bands are not always flat but may be bent or folded and are still considered gneissic banding even though the bands are not horizontal. The typical minerals seen in the dark colored bands are biotite micas and amphiboles, whereas the light-colored bands are typically quartz or light-colored feldspars. The protolith for gneiss can be any rock that contains more than one mineral, such as shale with its clay minerals, or an igneous rock with both dark-colored and light-colored silicate minerals, like granite. For gneissic foliation to develop, temperatures and pressures need to be quite high; for this reason, gneiss represents a high-grade of metamorphism.

Sometimes a metamorphic rock is seen with mostly amphibole minerals that define the foliation pattern. The resulting rock is known as an amphibolite. Occasionally, amphibolites also have layers of light-colored plagioclase minerals present; in this case, the rock is known as an amphibole gneiss. Amphibolites may not always have a foliation. The protolith for an amphibolite is typically a rock with a large amount of dark silicate minerals, such as a mafic or ultramafic igneous rock. Amphibolites require higher temperatures and pressures to form and are also high-grade metamorphic rocks.
Metamorphic rocks with schistose foliation exhibit layering of platy minerals, such as muscovite or biotite micas. Differential stress forces the realignment of the micas, such that their cleavage faces are oriented in the same direction; this results in a rock that sparkles as light is reflected off its minerals’ cleavage faces. The sparkly metamorphic rock is called schist, and typically the name will reflect which mica is present (Figure 13.10). By convention, when naming a metamorphic rock, the mineral in the lowest quantity is mentioned first: for example, garnet muscovite schist. The sedimentary rock shale is usually the protolith for schist; during metamorphism, the very tiny clay minerals in shale recrystallize into micas that are large enough to see unaided. Temperatures and pressures necessary for schistose foliation are not as high as those for gneiss and amphibolite; therefore, schists represent an intermediate-grade of metamorphism.

Metamorphic rocks with phyllitic foliation exhibit a layering of wavy platy minerals, such as muscovite or biotite micas; however, unlike schist, individual crystals are too small to be seen without magnification (Figure 13.11). The resulting metamorphic rock is called phyllite. Phyllite is a low-medium grade regional metamorphic rock in which the clay minerals and chlorite have been at least partly replaced by muscovite and biotite. This gives the surfaces of phyllite a satiny or phyllitic luster, brighter than the surface of slate (Figure 13.12). It is also common for the differential stresses under which phyllite forms to have produced a set of folds in the rock, making the foliation surfaces wavy or irregular, in contrast to the often perfectly flat surfaces of slaty cleavage.

The last example of foliation, slaty foliation (cleavage), is defined by the alignment of minerals too small to see, though foliation planes are still visible (Figure 13.12). The resulting metamorphic rock is called slate. Slate forms through low-grade metamorphism of a shale protolith. The clay sized minerals in the shale recrystallize into very tiny micas which are larger than the clay minerals yet still too small to be visible. Because these tiny micas are aligned, however, they control how the slate breaks, and the rock tends to break parallel to the mica alignment. In certain rare instances, some fossils from the original shale may be preserved and visible in slate. Slate has great economic value in the construction industry; due to its ability to break into thin layers and impermeability to water, slate is used as roofing tiles and flooring. In addition to the construction industry, slate helped transform classrooms when the slate blackboard (or chalkboard) was introduced. Has anyone ever moved a pool table? They’re so heavy because they are full of rock! Many have slate decks underneath the felt covering.

Foliated Metamorphic Rocks | |||
---|---|---|---|
Metamorphic Rock | Grain Size | Grade & Metamorphic Environment | Protolith |
Slate | Very fine |
Very low, 150°C-300°C (300-570°F) Regional |
Mudrock, shale |
Phyllite | Fine, with wavy layer and phyllitic luster |
Low, 300°C-450°C (570-840°F) Regional |
Mudrock, shale |
Schist | Medium to coarse |
Intermediate, 450°C-550°C (840-1020°F) Regional |
Mudrock, shale |
Gneiss | Coarse with light & dark bands |
High, >550°C (>1020°F) Regional |
Mudrock, granite, diorite |
What Is a Non-Foliated Metamorphic Rock?
Metamorphic rocks that lack foliation are referred to as non-foliated. This is primarily due to a lack of differential pressure involved during metamorphism. However, if the protolith rock is monomineralic (composed of one mineral type), such as limestone, dolostone, or sandstone, then a foliation will not develop even with differential pressure. Why? Calcite, dolomite and quartz sands are not platy minerals. These minerals will recrystallize into equant, coarse crystals, and the resulting metamorphic rock is named for its composition, not by foliation type.
Quartzite, is a metamorphic rock made almost entirely of quartz, for which the protolith was quartz sandstone (Figure 13.13). Because quartz is stable over a wide range of pressure and temperature conditions, few-to-no new minerals form in quartzite during metamorphism. Instead, the quartz grains recrystallize into a denser, harder rock than the original sandstone. If struck by a rock hammer, quartzite will commonly break right through the quartz grains, rather than around them as in quartz sandstone. Colors will vary, and a hardness test must be done to be sure the sample is quartzite. Quartzite is fairly tough and will sometimes be used decoratively in building stone, facades, or for kitchen or bathroom countertops.

Marble is a metamorphic rock made entirely of calcite or dolomite, for which the protolith was limestone or dolostone (Figure 13.14). Marble may have bands of different colors which were deformed into convoluted folds while the rock was ductile. Marble will typically be soft, and can be scratched with a steel nail, and if there is enough calcite present, will react (effervesce, fizz, bubble) when HCl is added. Marble is also used as decorative stone in buildings, or in countertops in homes. Italy has the famous Carrera Marble mine, which many sculptors, including Michelangelo, used for their great works. Additionally, many older grave markers, particularly those that appear blank or heavily worn, are likely composed of marble.

Anthracite coal (Figure 13.15) is much harder than the coal varieties we discussed in the Sedimentary Rock chapter. It is black in color with a submetallic luster. Anthracite accounts for <1% of coal mined in the US with most mined from northeastern Pennsylvania; it is the highest grade of coal, with around 86%–97% carbon content, which makes anthracite a hotter burning coal. Under higher temperatures and pressures the protolith, bituminous coal, loses more volatiles (water vapor), but the carbon content is enriched. This metamorphism is not recrystallization, as coal is mostly organic remains; however, due to changes in temperature and pressure, bonds are still broken and reformed.

Non-foliated Metamorphic Rocks | |||
---|---|---|---|
Metamorphic Rock | Dominant Composition | Grade & Metamorphic Environment | Protolith |
Quartzite |
Quartz Test: scratches glass (H>5.5) |
Intermediate to high Contact |
Sandstone |
Marble |
Calcite or Dolomite Test: Soft, will not scratch a copper piece (H=3); may react with HCl |
Low to high Contact | Limestone, dolostone |
Anthracite |
Carbon Test: Light heft, black color with a submetallic luster |
High Burial |
Bituminous coal |
Metamorphic Rocks of California
Most soapstones lack foliation and contain the mineral talc with lesser amounts of chlorite, pyroxenes, micas, amphiboles, carbonates, and other minerals (Figure 13.16). Recall that talc feels greasy and is incredibly soft. These properties are retained in the rock, and you will be able to feel the soapy-ness of the sample as well as scratch it with your fingernail. Typically, soapstone forms at subduction zones where heat, hot fluids (water), and directed pressure metamorphose protoliths like peridotites, dunites, and serpentinites. Many of the soapstones in California are found in the Santa Catalina Schist on Santa Catalina Island and the Sierra Pelona Schist near Palmdale.

Serpentinite also lacks foliation and is composed mainly of minerals from the serpentine group (Figure 13.17). Serpentinization occurs at subduction zones where heat, hot fluids (water), and directed pressure metamorphose peridotite or dunite. The name serpentinite alludes to the rocks mottled green, snake-skin appearance. This rock is significant because it is California’s state rock. Concentrations of serpentinite exist in the Franciscan Complex, Coast Ranges, the Trinity Ophiolite, portions of the Sierra Nevada and Klamath Mountains.

At subduction zones, the oceanic crust is relatively cool in comparison to the mantle, especially along its sea-floor upper surface. The metamorphism that takes place here is unique, with high-pressure but relatively low-temperature conditions. At these locations a mineral called glaucophane, which is blue in color, forms. This mineral is an important component of the rock known as blueschist (Figure 13.18).
As blueschist continues to subduct, it will eventually transform into eclogite at a depth of approximately 35 km (22 miles) (Figure 13.19). The eclogite will continue to sink deep into the mantle, never to be seen again because the rock will eventually melt. In only a few places in the world, where the subduction process has been interrupted by some other tectonic process, has partially subducted blueschist rock returned to the surface. One such place is the area around San Francisco; the rock here is known as the Franciscan Complex.


Metamorphic Rocks in California | |||
---|---|---|---|
Metamorphic Rock | Composition and Test | Metamorphic Environment & Grade | Protolith |
Serpentinite |
Serpentine Patchy green, waxy appearance, might scratch glass (H3-6) |
Low to intermediate Regional - Subduction zone, Hydrothermal |
Peridotite Dunite Serpentinite |
Soapstone |
Talc Soft (H=1), greasy feel; pearly luster |
Low to intermediate Regional - Subduction zone, Hydrothermal |
Dunite Peridotite |
Blueschist | Blue color | Low temperature, High pressure Regional - Subduction zone |
Basalt Gabbro |
Eclogite | Dense; may have garnet present; may be greenish in color | Low temperature, High pressure Regional - Subduction zone |
Basalt Gabbro |
Other Metamorphic Rocks
If a metamorphic rock is heated enough, it can begin to undergo partial melting in the same way that igneous rocks do. The more felsic minerals (feldspar, quartz) melt, while the more mafic minerals (biotite, hornblende) do not. When the melt crystallizes again, the result is light-colored igneous rock interspersed with dark-colored metamorphic rock. This mixed rock is called migmatite (Figure 13.20, left and left center).
Metaconglomerate (Figure 13.20, right center) looks similar to conglomerate, although sometimes the clasts are deformed (stretched or elongated). The original cement has recrystallized and may be quite durable. Some metaconglomerates may exhibit weak foliation, forming similar to slate or phyllite.
Hornfels (Figure 13.20, right) is a fine-grained non-foliated metamorphic rock with no specific composition, although it commonly forms from the protolith shale or mudrock. It is typically produced via contact metamorphic in the metamorphic aureole.

What Are the Different Metamorphic Environments?
Metamorphic environments are areas or regions where metamorphic rock forms. These environments experience varying conditions – amounts of heat and pressure – which can transform a protolith in to a metamorphic rock.
Large-Scale Metamorphic Environments
Regional Metamorphism: Most regional metamorphism takes place within the continental crust and is commonly associated with convergent plate boundaries and the production of mountains. Regional metamorphism is common during continental-continental convergence, like that which is forming the Himalayan Mountains in Asia, and at subduction zones, like in the Pacific Northwest, and also characterized what-would-become-California during the Mesozoic Era as the Sierra Nevada Mountains were forming (Figure 13.21, left). Commonly, regional metamorphism will produce foliated metamorphic rocks because there is directional pressure; for example, a clay-rich protolith will respond to the pressure by converting the clay to mica, which aligns to produce foliation. Increased temperature will also increase grain size and encourage the development of new index minerals.
Burial Metamorphism: Burial metamorphism occurs when sedimentary rocks are buried to depths of several kilometers (more than a mile), and temperatures greater than 300oC (570°F). Burial metamorphism overlaps, to some extent, with diagenesis, and grades into regional metamorphism as temperature and pressure increase. To the unaided eye, metamorphic changes may not be apparent at all. Rocks like anthracite and metaconglomerate typically are formed in this environment.
Hydrothermal Metamorphism: A variety of regional metamorphism, hydrothermal metamorphism occurs in oceanic crust near divergent boundaries. Here, the rocks are affected by hot, chemically reactive fluids. Typically, soapstone and serpentinite are produced because of hydrothermal metamorphism. During these metamorphic reactions’ fluids are released as chemical reactions occur and minerals alter. If a large amount of fluid, like that supplied by magma, is flushed through the system at high pressures and temperatures, it can substantially alter the chemical composition of the rock. This type of hydrothermal alteration is called metasomatism. A special type of metasomatism takes place where a hot pluton intrudes into carbonate rock such as limestone. Magmatic fluids rich in silica, calcium, magnesium, iron, and other elements can dramatically change the chemistry of the limestone, forming minerals that would not normally exist in either the igneous rock or limestone. A rock called skarn results, containing minerals such as garnet, epidote, magnetite, and pyroxene, among others.

Small-Scale Metamorphic Environments
Contact Metamorphism: Contact metamorphism occurs as the result of a temperature increase due to nearby magma body or lava flow. Recrystallization due to the increased temperature results in the formation of larger minerals, or sometimes in the formation of new minerals. The rocks closer in contact to the magma will form larger crystals due to the higher heat and may form high temperature index minerals such as garnet, staurolite, kyanite and sillimanite. The area surrounding the intrusion where the contact metamorphism effects are present is called the metamorphic aureole (Figure 13.21, right). Since differential pressures are not involved, contact metamorphism typically results in the formation of non-foliated rocks, like marble, quartzite, or hornfels.
Shock Metamorphism: While regional and contact metamorphism require significant time to pass for metamorphism to occur (up to several hundred thousand years), shock (impact) metamorphism takes place in a matter of seconds. This type of metamorphism occurs at the site of impact craters. Upon impact, the rocks in the vicinity are subjected to extreme stresses, and sometimes portions of these surface rocks become tiny blobs of melt that cool to form a type of glass called a tektite. Shock metamorphism is characterized by ultrahigh pressure conditions and low temperature. The resulting minerals (such as coesite and stishovite) and textures are characteristic of these conditions.
Fault Zone Metamorphism: Fault zone metamorphism affects rocks along geologic faults which are deformed due to pressures associated with shearing, compression, or extensional stresses, with minor changes due to heat. Shallow faults, those close to the earth’s surface and affected primarily by brittle deformation (<4 km or <2.5 mi), may grind the nearby rocks into smaller, angular fragments called incohesive fault breccia, or, if the breccia has been chemically altered, there may be a fine clay powder called incohesive fault gouge (Figure 13.22). Faults at depth, between 4-10 km (2.5-6 mi) will form cohesive fault breccia (cataclasite). Faults deeper than 10 km (>6 mi) will be affected by ductile deformation and mylonite is formed. The minerals in mylonitic rocks are deformed due to shear stresses associated with movement along the fault.
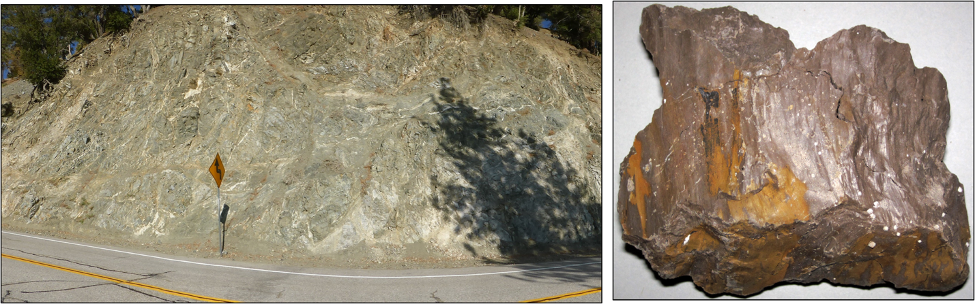
Attributions
- Figure 13.1: “The Rock Cycle” (CC-BY 4.0; Emily Haddad, own work)
- Figure 13.2: Derivative “Gray Shale” (CC-BY 2.0; James St. John via Flickr) and “Slate” (CC-BY 4.0; Chloe Branciforte, own work) by Chloe Brancifort
- Figure 13.3: “Confining/Differential Pressure” (CC-BY 2.0; Emily Haddad, own work)
- Figure 13.4: “Foliation” (CC-BY 4.0; Chloe Branciforte, own work
- Figure 13.5: Derivative of “Recrystallization” (CC-BY-SA 3.0; Karen Tefend via LibreText) by Chloe Branciforte
- Figure 13.6: “Index Minerals” (CC-BY 4.0; Chloe Branciforte, own work)
- Figure 13.7: “Metamorphic Grades” (CC-BY 4.0; Chloe Branciforte, own work)
- Figure 13.8: Derivative of “Gneiss 1” (CC-BY 2.0; James St. John via Flickr) and “Marble” (CC-BY 2.0; James St. John via Flickr) by Chloe Branciforte
- Figure 13.9: Derivative of “Gneiss 1” (CC-BY 2.0; James St. John via Flickr) and “Gneiss 3” (CC-BY 2.0; James St. John via Flickr) by Chloe Branciforte
- Figure 13.10: Derivative of “Mica Schist” (CC-BY 4.0; Chloe Branciforte, own work) and “Garnet Schist” (CC-BY 2.0; James St. John via Flickr) by Chloe Branciforte
- Figure 13.11: “Phyllite” (CC-BY 4.0; Chloe Branciforte, own work)
- Figure 13.12: Derivative of “Gray Slate” (CC-BY 4.0; Chloe Branciforte, own work) and “Red Slate” (CC-BY 2.0; James St. John via Flickr) by Chloe Branciforte
- Table 13.1: “Foliated Metamorphic Rocks” (CC-BY 4.0; Chloe Branciforte, own work)
- Figure 13.13: Derivative of “Blue Quartzite” (CC-BY 4.0; Chloe Branciforte, own work) and “Quartzite 3” (CC-BY 2.0; James St. John via Flickr) and “Quartzite 4” (CC-BY 2.0; James St. John via Flickr) and “Quartzite 1” (CC-BY 2.0; James St. John via Flickr) and “Quartzite 2” (CC-BY 2.0; James St. John via Flickr) by Chloe Branciforte
- Figure 13.14: “White and Blue Marble” (CC-BY 4.0; Chloe Branciforte, own work)
- Figure 13.15: “Anthracite” (CC-BY 4.0; Chloe Branciforte, own work)
- Table 13.2: “Non-foliated Metamorphic Rocks” (CC-BY 4.0; Chloe Branciforte, own work)
- Figure 13.16: “Soapstone” (CC-BY 4.0; Chloe Branciforte, own work)
- Figure 13.17: “Serpentinite” (CC-BY 4.0; Chloe Branciforte, own work)
- Figure 13.18: “Blueschist” (CC-BY 2.0; James St. John via Flickr)
- Figure 13.19: “Eclogite” (CC-BY 2.0; James St. John via Flickr)
- Table 13.3: “Unique Metamorphic Rocks of California” (CC-BY 4.0; Chloe Branciforte, own work)
- Figure 13.20: Derivative of “Hornfels” (CC-BY 2.0; James St. John via Flickr) by Chloe Branciforte
- Figure 13.21: Derivative of “Hornfels” (CC-BY 4.0; Steven Earle via OpenText) and “Contact Metamorphism” (CC-BY-SA 3.0; Jillcurie via Wikipedia) by Chloe Branciforte
- Figure 13.22: Derivative of “Gouge Created by San Andreas Fault” (CC-BY 2.0; Michael R. Perry via Flickr) and “Fault Gouge” (CC-BY 2.0; James St. John via Flickr) by Chloe Branciforte