2.9: Soils
- Page ID
- 14259
\( \newcommand{\vecs}[1]{\overset { \scriptstyle \rightharpoonup} {\mathbf{#1}} } \)
\( \newcommand{\vecd}[1]{\overset{-\!-\!\rightharpoonup}{\vphantom{a}\smash {#1}}} \)
\( \newcommand{\id}{\mathrm{id}}\) \( \newcommand{\Span}{\mathrm{span}}\)
( \newcommand{\kernel}{\mathrm{null}\,}\) \( \newcommand{\range}{\mathrm{range}\,}\)
\( \newcommand{\RealPart}{\mathrm{Re}}\) \( \newcommand{\ImaginaryPart}{\mathrm{Im}}\)
\( \newcommand{\Argument}{\mathrm{Arg}}\) \( \newcommand{\norm}[1]{\| #1 \|}\)
\( \newcommand{\inner}[2]{\langle #1, #2 \rangle}\)
\( \newcommand{\Span}{\mathrm{span}}\)
\( \newcommand{\id}{\mathrm{id}}\)
\( \newcommand{\Span}{\mathrm{span}}\)
\( \newcommand{\kernel}{\mathrm{null}\,}\)
\( \newcommand{\range}{\mathrm{range}\,}\)
\( \newcommand{\RealPart}{\mathrm{Re}}\)
\( \newcommand{\ImaginaryPart}{\mathrm{Im}}\)
\( \newcommand{\Argument}{\mathrm{Arg}}\)
\( \newcommand{\norm}[1]{\| #1 \|}\)
\( \newcommand{\inner}[2]{\langle #1, #2 \rangle}\)
\( \newcommand{\Span}{\mathrm{span}}\) \( \newcommand{\AA}{\unicode[.8,0]{x212B}}\)
\( \newcommand{\vectorA}[1]{\vec{#1}} % arrow\)
\( \newcommand{\vectorAt}[1]{\vec{\text{#1}}} % arrow\)
\( \newcommand{\vectorB}[1]{\overset { \scriptstyle \rightharpoonup} {\mathbf{#1}} } \)
\( \newcommand{\vectorC}[1]{\textbf{#1}} \)
\( \newcommand{\vectorD}[1]{\overrightarrow{#1}} \)
\( \newcommand{\vectorDt}[1]{\overrightarrow{\text{#1}}} \)
\( \newcommand{\vectE}[1]{\overset{-\!-\!\rightharpoonup}{\vphantom{a}\smash{\mathbf {#1}}}} \)
\( \newcommand{\vecs}[1]{\overset { \scriptstyle \rightharpoonup} {\mathbf{#1}} } \)
\( \newcommand{\vecd}[1]{\overset{-\!-\!\rightharpoonup}{\vphantom{a}\smash {#1}}} \)
\(\newcommand{\avec}{\mathbf a}\) \(\newcommand{\bvec}{\mathbf b}\) \(\newcommand{\cvec}{\mathbf c}\) \(\newcommand{\dvec}{\mathbf d}\) \(\newcommand{\dtil}{\widetilde{\mathbf d}}\) \(\newcommand{\evec}{\mathbf e}\) \(\newcommand{\fvec}{\mathbf f}\) \(\newcommand{\nvec}{\mathbf n}\) \(\newcommand{\pvec}{\mathbf p}\) \(\newcommand{\qvec}{\mathbf q}\) \(\newcommand{\svec}{\mathbf s}\) \(\newcommand{\tvec}{\mathbf t}\) \(\newcommand{\uvec}{\mathbf u}\) \(\newcommand{\vvec}{\mathbf v}\) \(\newcommand{\wvec}{\mathbf w}\) \(\newcommand{\xvec}{\mathbf x}\) \(\newcommand{\yvec}{\mathbf y}\) \(\newcommand{\zvec}{\mathbf z}\) \(\newcommand{\rvec}{\mathbf r}\) \(\newcommand{\mvec}{\mathbf m}\) \(\newcommand{\zerovec}{\mathbf 0}\) \(\newcommand{\onevec}{\mathbf 1}\) \(\newcommand{\real}{\mathbb R}\) \(\newcommand{\twovec}[2]{\left[\begin{array}{r}#1 \\ #2 \end{array}\right]}\) \(\newcommand{\ctwovec}[2]{\left[\begin{array}{c}#1 \\ #2 \end{array}\right]}\) \(\newcommand{\threevec}[3]{\left[\begin{array}{r}#1 \\ #2 \\ #3 \end{array}\right]}\) \(\newcommand{\cthreevec}[3]{\left[\begin{array}{c}#1 \\ #2 \\ #3 \end{array}\right]}\) \(\newcommand{\fourvec}[4]{\left[\begin{array}{r}#1 \\ #2 \\ #3 \\ #4 \end{array}\right]}\) \(\newcommand{\cfourvec}[4]{\left[\begin{array}{c}#1 \\ #2 \\ #3 \\ #4 \end{array}\right]}\) \(\newcommand{\fivevec}[5]{\left[\begin{array}{r}#1 \\ #2 \\ #3 \\ #4 \\ #5 \\ \end{array}\right]}\) \(\newcommand{\cfivevec}[5]{\left[\begin{array}{c}#1 \\ #2 \\ #3 \\ #4 \\ #5 \\ \end{array}\right]}\) \(\newcommand{\mattwo}[4]{\left[\begin{array}{rr}#1 \amp #2 \\ #3 \amp #4 \\ \end{array}\right]}\) \(\newcommand{\laspan}[1]{\text{Span}\{#1\}}\) \(\newcommand{\bcal}{\cal B}\) \(\newcommand{\ccal}{\cal C}\) \(\newcommand{\scal}{\cal S}\) \(\newcommand{\wcal}{\cal W}\) \(\newcommand{\ecal}{\cal E}\) \(\newcommand{\coords}[2]{\left\{#1\right\}_{#2}}\) \(\newcommand{\gray}[1]{\color{gray}{#1}}\) \(\newcommand{\lgray}[1]{\color{lightgray}{#1}}\) \(\newcommand{\rank}{\operatorname{rank}}\) \(\newcommand{\row}{\text{Row}}\) \(\newcommand{\col}{\text{Col}}\) \(\renewcommand{\row}{\text{Row}}\) \(\newcommand{\nul}{\text{Nul}}\) \(\newcommand{\var}{\text{Var}}\) \(\newcommand{\corr}{\text{corr}}\) \(\newcommand{\len}[1]{\left|#1\right|}\) \(\newcommand{\bbar}{\overline{\bvec}}\) \(\newcommand{\bhat}{\widehat{\bvec}}\) \(\newcommand{\bperp}{\bvec^\perp}\) \(\newcommand{\xhat}{\widehat{\xvec}}\) \(\newcommand{\vhat}{\widehat{\vvec}}\) \(\newcommand{\uhat}{\widehat{\uvec}}\) \(\newcommand{\what}{\widehat{\wvec}}\) \(\newcommand{\Sighat}{\widehat{\Sigma}}\) \(\newcommand{\lt}{<}\) \(\newcommand{\gt}{>}\) \(\newcommand{\amp}{&}\) \(\definecolor{fillinmathshade}{gray}{0.9}\)2.9.1 Introduction
Along with oxygen and water, soils are essential to human existence. Almost all of our food comes, directly or indirectly, from crops grown in the soil. The only significant exception, of course, is food from the oceans. Hydroponically grown crops are an insignificant exception—and the infrastructure and cost involved in hydroponic agriculture are likely to doom it forever to a minor role in human food supply. All of our lumber, most of our paper products, and much of our clothing ultimately come from the soil. Our dependence on soils will continue, but the world stock of soils cannot increase. In fact, it is decreasing, because of soil erosion, as well as urbanization (and suburbanization). As our reserves of fossil fuels continue to shrink, energy resources from biomass (living plant material) are likely to increase.
The meaning of the term “soil” varies greatly depending upon the field of endeavor in which it is used. Agriculturalists use the term narrowly to mean the uppermost layer of surface deposits, in which plants grow and agriculture is practiced. The soil scientist has a somewhat wider view: soil is the uppermost layer of surface deposits, almost always extending well below the agriculturalist’s soil, which has developed upon underlying material by the influence of various soil-forming processes acting over long times. The geologist has an even broader view of soils as surface materials developed by weathering processes that have broken down bedrock into regolith, and the civil engineer (who practices the discipline of soil mechanics, and who is responsible for the siting and design of structures with foundations) takes a similarly wide view of soil as all materials at the surface that can be excavated without blasting. In the following sections, we’ll look at the nature of soils mainly from the standpoint of the soil scientist (what is the nature of soils, how do they develop, and how are they classified?).
The study of soils as natural bodies is called pedology (and its practitioners call themselves soil scientists, or pedologists). You can also think in terms of the pedosphere. (There is a tendency among science educators nowadays to think in terms of “spheres”: geosphere, hydrosphere, biosphere, atmosphere, cryosphere. These terms are useful, in a broad and loose sense, to describe zones or domains of related materials and processes. I think that the suffix “-sphere” began to be used because in a very general sense these domains are like spherical shells around the Earth. I myself think that “sphere-ology” tends to be overdone on the part of the “spherophiles”, but it remains a useful pedagogical device.)
Soils can be thought about and studied on a wide range of scales: from large regions of continents, in the context of major soil types, to the distribution of soils in a local area of varied topography and substrate composition (Figure 2-23A), to the vertical profile of soil at a given point on the land surface (Figure 2-23B), to the texture and structure of the soil at a point in such a profile (Figure 2-23C), to the details of physics, chemistry, and biology of the soil on the colloidal and atomic scale (Figure 2-23D).
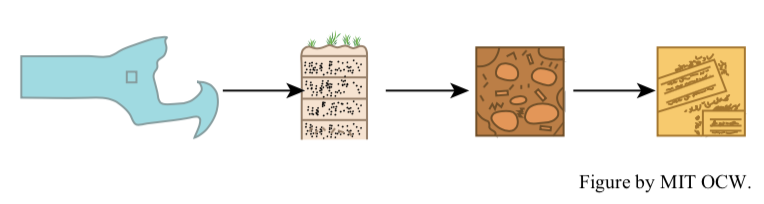
Soils have a great variety of constituents. Here is a list of the most important kinds of soil constituents:
- mineral particles
- colloids
- organic matter
- microorganisms
- soil solutions
- soil gases
Most soils consist mainly of mineral particles. Such soils are often called mineral soils. Some soils, however, consist mostly of organic matter. In general, soils contain the same kinds of mineral particles as do sediments; go back and review the section on sediments above. Most of the soils you are likely to encounter consist mainly of quartz, plus variable percentages of potassium feldspar and rock fragments, in the coarse fraction, and mainly particles of clay minerals, iron oxides, and aluminum oxides, in the fine fraction. The remaining constituents in the list above are described in the following sections.
2.9.2 Colloids
Colloids are mixtures of materials in which one substance exists as extremely small particles, called colloidal particles, which are dispersed in a second substance, which you can think of as the medium. In the case of colloidal particles in a uniform liquid or gaseous medium, the term colloidal solution is commonly used. In the context of soils, we’re dealing with small particles consisting of minerals or organic matter that are dispersed in the aqueous pore solutions in the soil or are aggregated together in great numbers, or cling to the larger soil particles. Colloids are important in a great many materials, both natural and manufactured.
Most clay-mineral particles have a platy shape, and they have a tendency to stick together in aggregates of many particles, held together by certain short-range electrostatic forces. Such forces are small, but so are the particles— and it’s important to realize that because the ratio of surface area to volume increases with decreasing particle size, clay-mineral particles are in a sense “almost all surface and very little volume”. The state of aggregation of the clay- mineral fraction is widely variable, and the macroscopic strength and consistency properties of the deposit vary greatly corresponding to that. Also, as you will soon see, the small size of the particles, together with the electrical charges characteristic of their surfaces, make the tiny particles chemically extremely reactive.
Colloidal particles are in a kind of “no person’s land” between true solutions and undoubtedly solid particles. At one extreme are ions and molecules in an aqueous solution. The smallest ions are of the order of atomic dimensions, about 0.1 nanometers (that is, about 10-10 meters). Many molecules that consist of a large number of atoms are much larger, but they still behave like smaller ions in true solutions. At the other extreme are particles larger than a few micrometers, which is large enough to be seen with light microscopes. Such particles are large enough to settle in the surrounding fluid under the influence of gravity.
Typical soils contain several important kinds of colloids. Here’s a briefly annotated list of the most important:
- clay-mineral particles: There are several types; see the earlier background section on clay mineralogy. These types differ in their physical and chemical properties, partly because of their differing size but mainly because of their differing surface charges and their differing susceptibility to take on or release positive ions and water molecules to and from their internal surfaces between the TOT layers.
- iron and aluminum oxides: These are especially important in the highly weathered soils of warm and humid regions, where silicate minerals are broken down further to oxides. The main aluminum oxide is gibbsite, and the main iron oxides are goethite and ferrihydrite (no details of mineralogy here).
- humus: These are especially abundant in the upper parts of soils, nearest the surface. Humus colloids are not crystalline structures: they are complex molecular chains and rings of carbon atoms bonded to hydrogen, oxygen, and nitrogen atoms. They are among the smallest of colloidal particles in soils. They have an enormous capacity to adsorb water onto their surfaces.
The great importance of soil colloids arises from their chemical reactivity. There are two reasons for that: surface area, and surface charge. The following elaborates on those points.
- surface area: Chemical reactions between a solid and the fluid with which it is in contact take place at the surface of the solid, at the interface between the solid phase and the liquid phase. The rate of such a chemical reaction depends upon a number of important factors, aside from the nature of the reaction itself. Temperature is one of those important factors: in general, the higher the temperature, the faster the reaction. That’s because the energy of thermal vibration of the atoms and molecules in both the solid phase and the liquid phase increases with temperature. Also important, however, is the area of the surface at which the reaction takes place, per unit volume of the reacting system. The extremely small size of colloidal particles means that their surface area, per unit bulk volume of material, is spectacularly large.
- surface charge: At any given tiny region inside a mineral particle, the electric charges associated with the ions of the structure balance out. At the surface of the particle, however, the picture is different—because the ions right at the surface are bonded only to the ions below them. That means that there is a kind of “unsatisfied” electric charge at the surface of the particle. With regard to clay-mineral particles, the surface charge is generally negative on the broad faces of the exposed TOT sheets and positive along the narrow edges of the plates. For macroscopic mineral particles, like sand grains, these surface charges are almost entirely negligible, but when the particles are extremely small their enormous area-to-volume ratio makes these surface charges very important.
Take a nostalgia trip back to secondary-school math and think about the formulas for the volume and the surface area of some regular geometric solid. A sphere is a good example. The volume of a sphere is (1/6)πD3, where D is the diameter of the sphere. In scientific parlance, the volume goes as the cube of the diameter. The surface area of the sphere is \(πD^2\), so the surface area goes as the square of the diameter.
What’s important here is not the particular formulas, or the values for volume and area of a particular sphere they give, but how the ratio of surface area to volume varies with the diameter of the sphere. You can easily see from the two formulas given above that the ratio of surface area to volume of any sphere is [πD2]/[(1/6)πD3], or, simplifying, 6/D. Ignore the factor 6 there; what’s important is the 1/D part. The ratio of surface area to volume goes as 1/D. That means that as the diameter decreases, the ratio of surface area to volume gets bigger.
Now for a revealing example of this 1/D effect. Think about a sphere 10 cm in diameter—about the size of a softball, a grapefruit, or a ball of pizza dough. It has a surface area of about 0.0314 square meters (or, in scientific notation, 3.14 x 10-2 m2). Now compare that with a sphere 1 mm in diameter. That sphere has a surface area of only 3.14 x 10-6 m2, far smaller, by a factor of ten thousand. But suppose you took the entire volume of the 10 cm sphere and converted it into an equivalent volume of 1 mm spheres. With some further simple math, and using the formula for the volume of a sphere, you would determine that the 10 cm sphere is equivalent to 106 (that’s one million) of the little ones. Now the total surface area of the little ones is (106)(3.14 x 10-6) m2, or 3.14 x 100 m2. That doesn’t sound like a lot, but compare it with the surface area of the original 10 cm sphere, which was 3.14 x 10-2 m2. We see, then, that the aggregate area of the little spheres is a hundred times the area of the volume-equivalent big sphere! This is a concrete example of the 1/D effect: we reduced the diameter of the sphere by a hundred (ten centimeters to one millimeter), and the surface area of the equivalent volume increased by a factor of a hundred!
The combination of surface area and surface charge is a potent one. We need to deal with two different but related effects: coagulation, and ion exchange. Figure 2-24 is a cartoon that shows the various phenomena discussed in the following paragraphs.
coagulation: Clay-mineral particles, with their negative charges on faces and positive charges on edges, tend to clump together by electrostatic attraction, in an edge-to-face arrangement. (Keep in mind that like charges repel and unlike charges attract.) This tendency for coagulation is one of the important factors that make clay-rich soils sticky and tenacious.
ion exchange: As you will see in a later chapter, on water, the water molecule has a positively charged “side” and a negatively charged “side”. Because of that, water molecules tend to be attracted to the charged surfaces of the colloidal particles. Picture a swarm of water molecules, perhaps up to several molecules thick, at the clay-particle surface, jiggling around and jostling one another, now and then trading places with other water molecules from the solution beyond. That layer of water molecules acts as a kind of “cushion” for the particle. Included in the layer of loosely bound water molecules are positive ions, which are attracted to the negatively charged faces of the clay particles. Also, smectite clays take up and give off water and positive ions to and from their internal surfaces. These loosely bound water molecules are the last to be extracted by plant roots as a soil dries between rains. You know that potassium is one of the most important plant nutrients. Its continued abundance in the soil is largely the result of its attraction to colloids, together with recycling in organic matter. Because of this combination of attraction to colloids and recycling by plants, under natural conditions the storehouse of available potassium, released by chemical weathering of primary minerals, is maintained at a workable level for plant growth in the face of continuing loss in solution to downward-percolating water from the surface.
2.9.3 Organic Matter
The uppermost parts of surface deposits—the soil of the soil scientist and especially of the agriculturalist—usually has an abundance of organic matter as well as inorganic mineral matter. It’s not easy to specify what’s meant by organic matter. It comprises a wide and complex variety of chemical compounds that a chemist would call organic compounds. Organic compounds are those that consist of carbon atoms, bonded to one another and to atoms of other elements, mainly oxygen and hydrogen but including such elements as nitrogen and sulfur as well, in a great variety of ways. Organic compounds are both natural and laboratory-synthesized.
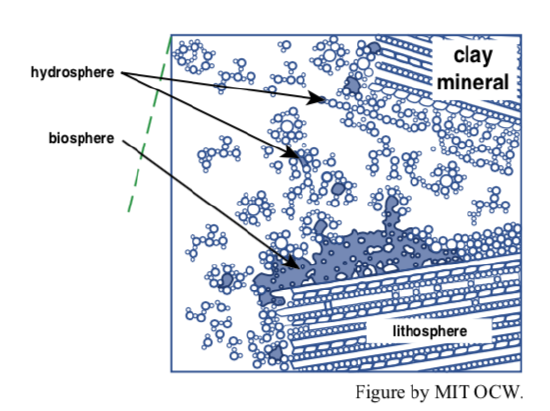
The organic matter of soils is ultimately derived from plant growth. (Living plants and animals are not conventionally considered to be organic matter: you have to wait until the organism dies!) Nonliving plant tissues themselves are organic matter, as are the great variety of organic compounds that result from partial decay of that plant material. The tissues of dead animals and their decay products also represent soil organic matter, although not nearly in the same abundance as plant-derived organic matter.
A deep understanding of soil organic matter would necessitate a much greater familiarity with organic chemistry (the branch of chemistry that deals with organic compounds) than is appropriate for this course. Here we can only touch upon some of the most important considerations and kinds of materials.
2.9.3.2 The Role of Soil Organic Matter in the Carbon Cycle
The element carbon is a key substance in the workings of the Earth’s near-surface environment. That comes about because of its important role in living organisms, soil organic matter, the atmosphere and the oceans in the form of gaseous and dissolved carbon dioxide, and carbonate sediments. There will be a more detailed treatment on the Earth’s carbon cycle in a much later chapter on geochemical cycles. It must suffice here to point out that the soil plays a major role in that carbon cycle. It’s been estimated that the Earth’s soil contains almost twice as much carbon as the stock of living vegetation and the atmosphere combined.
Plants take up carbon dioxide gas from the atmosphere and convert it, by sunlight-powered photosynthesis, into the organic compounds that constitute plant material. When the plants die, some of the dead plant material is decomposed back to carbon dioxide and water by oxidation. In part that decomposition involves non-biological chemical reactions, but in great part it is mediated by the metabolic activity of soil microorganisms. The end result, however, is the same: production of CO2. Some of that CO2 remains dissolved in soil water and some is released into the atmosphere.
2.9.3.3 Kinds of Soil Organic Matter
There are many kinds of organic matter. These compounds range from easily decomposable to very difficultly decomposable. A typical plant consists of easily transformed compounds like polysaccharides and lipids, stiffened with the much more resistant materials cellulose and lignin, the principal components of woody plant tissue. Lignins, of which there are various kinds, are the slowest plant tissues to decompose. Only a few kinds of organisms, mainly fungi, can break down lignins.
The ultimate residue left behind by breakdown of plant materials is called humus. What’s called humus comprises a number of very stable organic compounds. Humus is always dark in color, the reason being that the chemical structures in the humus absorb nearly all wavelengths of visible light. Humus is so fine grained that its particles (which in reality are large molecules) are at the fine end of the colloidal size range. These compounds, whose basic building blocks are carbon hexagonal ring structures (called aromatic compounds, in organic chemistry) with large molecular weights (that is, containing very large numbers of polymerized atoms in their structures). They are the organic materials that are the most resistant to microbial decomposition. The water-holding capacity of humus, mentioned in an earlier section, is several times greater than that of clay-mineral particles.
Decomposition of humus is a slow process. The typical half-life of humus (that is, the time it takes to decompose half of an initial mass of the material) is measured in many decades, or even a few centuries! An important effect that slows the decomposition is complexing of the molecules of the humus with inorganic clay-mineral particles. By poorly understood processes, this association protects the humus from decay.
2.9.3.4 The Role of Organic Matter in Soils
With respect to plant growth, humus is an essential soil component. It’s common knowledge among gardeners that plants grow better in soils rich in humus. There are various reasons for that, both direct and indirect. It’s not just a matter of supply nutrients: humus is not particularly rich in nutrients (despite what many organic gardeners have been led to believe). Most of the nutrients needed by plants are inorganic ions dissolved in aqueous soil solutions, although humus does play some role in the nutrient supply for plants.
It’s the indirect effects of humus on soil properties that are the most beneficial aspects of humus. Humus can be viewed as a soil conditioner. These effects involve physical, chemical and biological properties of the soil In terms of physical effects, humus reduces the stickiness and cohesion of clay-rich soils, and it promotes the formation of granular texture, so prized by agriculturalists because it enhances the tillability of the soil. In terms of chemical effects, humus is even more effective at adsorbing and holding onto nutrient ions than are clay-mineral particles. In terms of biological effects, humus is the most important substrate for the life activities of soil microorganisms; it provides most of the food for detritus-feeding soil organisms.
If you are willing to be overwhelmed by the multitude of ways that organic matter affects soil properties, plant productivity, and environmental quality, look at Figure 2-25.
2.9.4 Soil Organisms
The last really important component of the deposit comprises soil organisms: the plants and animals that spend part or all of their lives in the soil. The soil is one of the Earth’s most important ecosystems. (For a brief brush-up on ecology and ecosystems, see the background section below.) Every handful of soil contains literally billions of organisms—almost all of them microorganisms, too small to be seen with the unaided eye. The microbiology of soils is a fully developed and very important discipline in itself, and I can only touch upon it here.
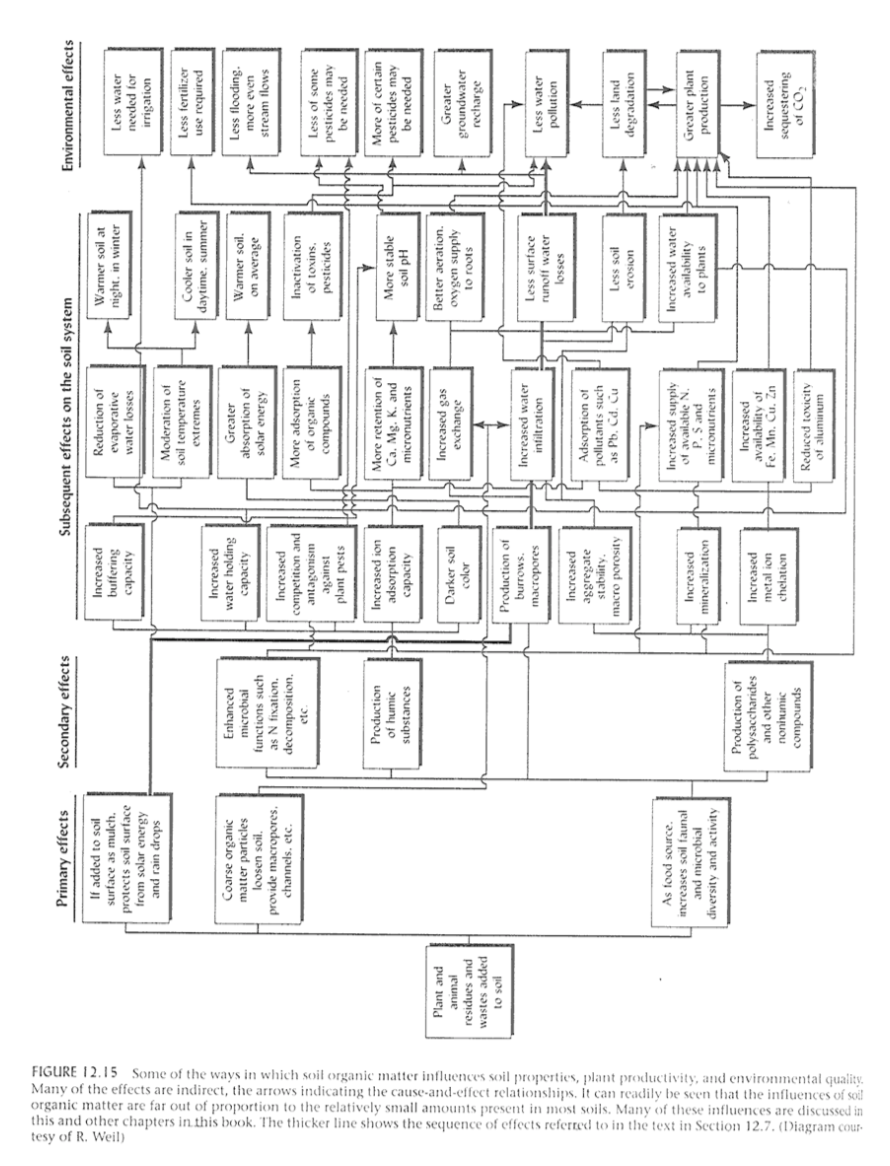
1. The term ecology, which tends to be used too loosely in the popular media these days, is the study of the interrelationships between organisms and their environment. The largest entity studied in ecology is the ecosystem, which consists of some chosen part of the physical and chemical environment and all of the organisms within it. An ecosystem involves all of the physical, chemical, and biological processes and interactions that operate within the given part of the environment. An ecosystem can be as large as the entire biosphere or as small as a puddle of water at our feet.
2. What are the elements of an ecosystem? A habitat is the local environment in which a given organism lives. Ecosystems usually involve a number of different and distinctive habitats. A similar but different concept is that of an ecological niche, which is the position of the organism in its habitat, including its way of life and its role in the ecosystem. Most habitats are occupied by several species, each with its own ecological niche. Each species in an ecosystem is represented by a number (usually, but not always, large) of individual organisms, called a population. A population of two or more species that occupy a given habitat is called a community. There may be more than one community in a given ecosystem.
3. Within any ecosystem, there typically are many kinds of interactions among constituents, both living and nonliving. These interactions can be viewed in terms of flows of matter and energy through the ecosystem. Organic compounds are synthesized from the environment by producers, which in all but the most specialized ecosystems are photosynthesizing plants. The producers are in turn consumed by plant-eating animals, called herbivores. Some of the herbivores are in turn consumed by carnivores (or by omnivores, which each both plants and animals). Other elements of the ecosystem are parasites, which feed on living organisms without killing them, and scavengers, which feed on dead organisms.
4. The sequence of species ranging from the producers, at one end, to carnivores that no other carnivores eat, at the other end, is called a food chain. Often the term food web is more appropriate, because the actual situation is more complicated than a simple linear arrangement of species. The tissues of producers, herbivores, and carnivores that are not consumed by species higher in the food chain are broken down by organisms called decomposers, which are usually bacteria.
5. When we think of ecosystems, we tend to think of tropical rainforests, or broad expanses of grasslands on which large mammals roam around and get photographed by ecotourists. In a real and important sense, however, a shovelful of natural soil is an ecosystem, and a very complex one at that.
One way of classifying soil organisms is into plants and animals. There’s more to that than it might seem, however, because although we all learned, when we were small, that life is divided into an animal kingdom and a plant kingdom, most biologists these days recognize five kingdoms. But that’s another story we need not deal with in this course. There are two more useful ways, for us, of classifying soil organisms:
- heterotrophs vs. autotrophs: autotrophs are organisms that generate their own tissues from inorganic raw material, by use of (mainly) solar energy. Plants are autotrophs. Heterotrophs are organisms that use organic matter produced by other organisms as food.
- soil organisms can be classified as macroorganisms (organisms with size ranging down to that of, say, earthworms), mesooorganisms (organisms ranging down to a small fraction of a millimeter), and microorganisms (organisms of microscopic size, smaller than about a tenth of a millimeter).
The numbers of kinds of organisms per unit volume of a soil increases strongly with decreasing organism size. A typical soil might contain several species of vertebrates and several species of earthworms, along with as many as several tens of species of insects, dozens of species of mesoorganisms, but thousands of species of microorganisms. Moreover, the numbers of organisms per unit volume increases spectacularly with decreasing size: although, obviously, numbers vary widely depending upon the type and condition of the soil, numbers of macroorganisms and mesoorganisms are large but not overwhelming, whereas the numbers of microorganisms, per cubic meter, say, might be in the trillions!
A whole chapter should be devoted here to the nature and significance of the life activities of soil organisms. Interesting as they are, the activities of what have been called ecosystem engineers (vertebrate burrowers, earthworms, ants, termites, and the like) in modifying the physical nature of the soil pale in comparison with the life activities of soil microorganisms. Microorganisms play the essential role of decomposing soil organic matter (mainly plant residues) and converting it to carbon dioxide, water, dissolved ions, and the ultimately resistant residues called humus earlier in this section.
Several major kinds of microorganisms are well represented in soils. The most important are algae, bacteria, and fungi, and various kinds of protozoans. Algae, which are photosynthetic plants, are abundant only in the uppermost parts of deposits, very near the sunlit surface. Fungi and protozoans are important but restricted to the shallow zones. Bacteria, on the other hand, range widely downward to great depths.
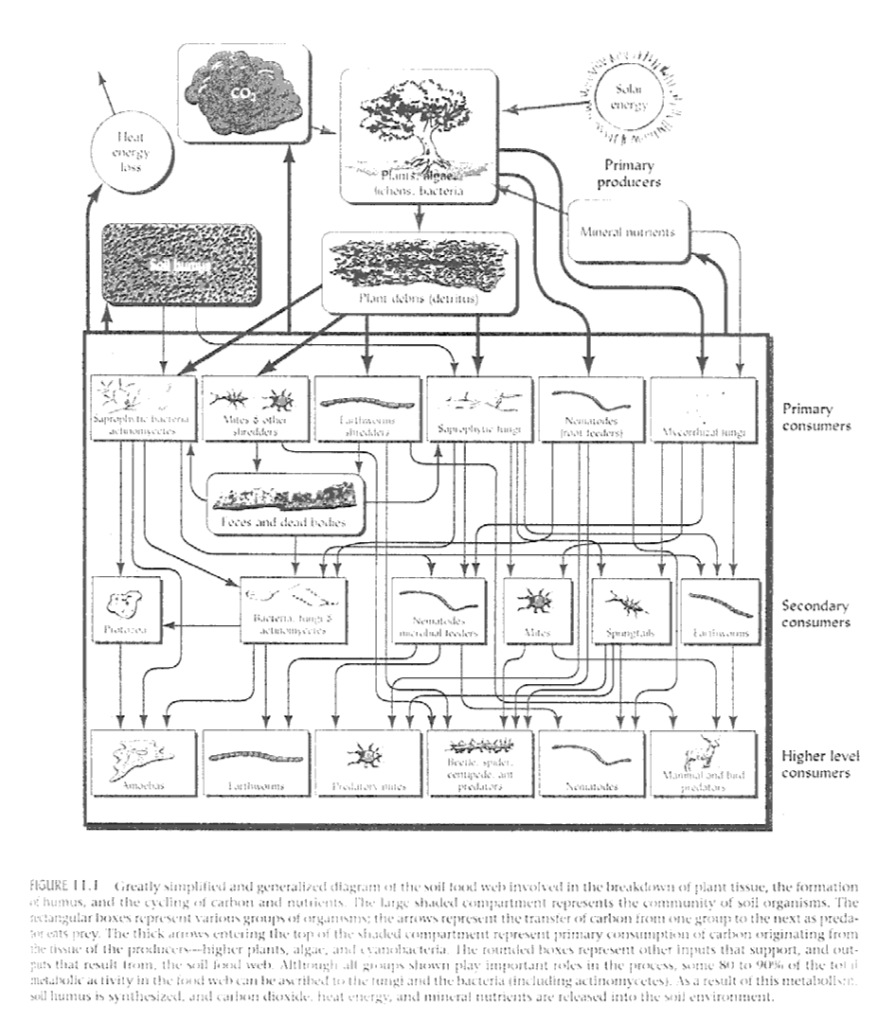
Figure 2-26 shows a diagram of the soil food web. I’ve preserved the original caption for you. Note that it’s described as “greatly simplified and generalized”!
2.9.5 Soil Solutions and Soil Gases
All soils are porous to some extent (that is, they contain empty spaces, called pore spaces or void spaces, among the various solid constituents). It stands to reason that the pore spaces must contain either a gas or a liquid, or some of both. Whether the pore spaces are occupied by solely by liquid or whether they are occupied mainly by a gas depends upon where the region of soil lies relative to the groundwater table: below the groundwater table the soil is fully saturated with an aqueous pore solution, whereas above the groundwater table the pore spaces are occupied mainly by gas.
The reason I have used the term “aqueous pore solution” rather than just “pore water” is that the water in the pore spaces contains a great variety of dissolved substances, in varying concentrations. Just after a rainstorm, as the rainwater percolates downward to replenish the water table, the concentrations of dissolved materials is very low (except for dissolved atmospheric gases), but as time goes on some of the constituents of the soil particles are leached out to go into solution. The chemistry of such soil solutions is a important part of soil science.
Above the groundwater table, the pores spaces are filled mainly with gas. The composition of soil gases is not greatly different from that of the atmosphere, with one major exception: owing to continuing decay of organic matter, the concentration of carbon dioxide is typically substantially higher than in the overlying atmosphere.
Even in the soil zone above the groundwater table, the soil is seldom entirely dry: some residual water clings tenaciously to the soil particles. I will defer discussion of that until the chapter on groundwater.
2.9.6 Processes of Soil Development
The development of a soil (in the agriculturalist’s and soil scientist’s sense) depends upon five major (and interacting) factors, as shown in Figure 2-27. Of these, you might think that the parent material is the most important. It’s generally believed, however, that climate is even more important than parent material. The relative importance of climate and parent material depends to a great extent upon time of development: in the initial stages of soil development, parent material is certainly the more important, but, as time goes on, soils developed upon different parent materials in a given climate tend to become more and more similar. Obviously, important differences as a function of parent material must remain: if for one soil the parent material is rich in quartz, and for another soil the parent material is a limestone or a mafic igneous rock like basalt, the soils can never converge.
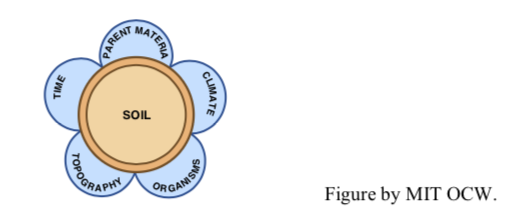
Topography is generally of secondary importance; its role lies largely in governing the downslope creep of already developed soil, which tends to “freshen” the uppermost layer of the surface deposit by exposing less weathered material beneath.
The importance of organisms lies in their ability to add the all-important organic matter to the soil and to process that organic matter back into inorganic compounds, including nutrients for yet more plant growth.
I might also point out that the five factors are not entirely independent of one another; for example, organisms depend strongly on climate, and topography depends quite strongly upon climate as well, and also upon parent material and time.
This is a good place to point out that some soils develop directly upon bedrock, but in many if not most cases, soil develops upon surface deposits, called sediments earlier in this chapter, that were derived from bedrock somewhere else and transported to the site of soil formation. In some places, the surface deposit is grossly “out of place”. That’s true of almost all glaciated areas, New England being no exception. It’s also true of areas of deposition from streams and rivers. In such areas, soil formation is usually nowhere near the “ultimate” or “equilibrium” soil, of the kind you would find in a low-latitude, non-glaciated area of low relief, where bedrock is weathered very deeply over a very long period of geologic time and the soil is highly developed.
(But just because a soil has had a long time to develop doesn’t mean that it will be rich and deep: in the rain forests of the humid tropics, intense leaching removes nutrients released by chemical weathering so rapidly that the soil is thin, and has only a thin layer rich in organic matter at the surface; the lush stand of plants is in tenuous existence, recycling nutrients very efficiently. When such vegetative cover is removed, the soil rapidly becomes irreversibly barren.)
A variety of physical and chemical processes act to develop soil from fresh regolith. One of the most important of these we have already dealt with: weathering of preexisting bedrock and/or further weathering of relatively fresh and unweathered surface deposits. Another important process is vertical transport of matter in either dissolved or colloidal form by upward or downward flow of soil water. Such processes come under the soil scientists’ term translocation. Translocation also includes vertical mixing or churning by macroorganisms, most importantly earthworms and certain kinds of insects. Biological processes, most importantly humification of plant material, and chemical and organism-aided processes of cycling of nutrients and other compounds (most importantly nitrogen and carbon) are further soil-forming processes.
The nature of water flow in the vertical direction in the soil, which is largely a function of climate (intensity and frequency of rainfall; the effect of temperature, humidity, and vegetative cover upon evaporation from the soil), determines whether matter in solution undergoes net downward transport or net upward transport in the soil. This is the aspect of climate that’s most important in soil development, along with the nature and rate of growth of vegetation.
2.9.7 Soil Profiles
Soil scientists classify soil on the basis of the sequence and nature of vertical zones or layers, called horizons, in the soil. Each of the enormous variety of soil types has a characteristic succession of such horizons, each with its thickness, composition, physical properties, and transitions to overlying and underlying horizons. The particular succession of horizons is called a soil profile.
To have a good picture of a soil profile, you need to go into your backyard and dig a trench, carefully, about a meter deep and wide enough for a good side view, with one side planar and almost vertical and shaved off carefully for good viewing with minimal smearing of soil material on the viewing face. Excavations for foundations, or even big holes for planting of trees or shrubs, can serve the purpose well. For the best and most representative results, you need to be sure to dig at a spot that hasn’t been filled or excavated in recent times, by humans, either before or after the time of European settlement, and hasn’t been cultivated, either. In New England, woodlands that served as pasture land rather than as tilled fields are the best places to see undisturbed soil profiles.
It’s dangerous for me to describe one or a few soil profiles for you, because there is such a multiplicity of important soil types (see the next section), but for concreteness, and a good start in thinking about soil horizons and classification, here’s a description of a typical New England soil (Figure 2-28). The two conditions such a soil experiences that are the most important factors in its development are (1) strong net downward movement of water through the profile, and (2) acidic waters. In the traditional classification of soils, such a soil would be called a podzol soil.
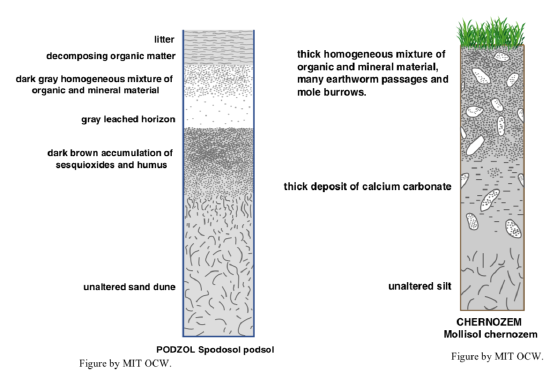
Right at the surface is a layer of forest litter: leaves and twigs that have fallen recently, within the last few years. That grades down into a layer of organic matter in a progressively more advanced state of humification with depth. Together these uppermost layers, called the H horizon if usually water-saturated or the O horizon if not usually water-saturated, are the part of the soil that’s by far the richest in organic matter and living organisms—and most conducive to cultivation, in which case the original organic-rich layer is tilled and mixed downward to form the best medium for plant growth. Its thickness commonly ranges from just a few centimeters upward to ten to twenty centimeters. It passes rather abruptly into a layer consisting mainly of mineral grains, usually mostly quartz (if the soil is well developed and the parent material had a fairly high percentage of quartz), with a characteristic light gray color. Much, or most, or in extreme cases even all, of the other silicate minerals have been leached out of this layer by decomposition by net downward passage, from the surface, of waters rendered acidic by (1) dissolved CO2 from the atmosphere and (2) organic acids produced in the uppermost organic-rich layer. This horizon is an example of what’s called the A horizon. The A horizon passes downward, again fairly abruptly, into a layer, called the B horizon, with a characteristic color, usually combinations of yellow or orange or red or brown. Here is where iron leached out of the material of the A horizon is reprecipitated as hydrous iron oxides within the pore spaces among the existing mineral grains, and fine clay-mineral particles produced by weathering reactions in the A horizon have been deposited. (The general term for mobilization and removal of such things as iron and aluminum from a soil horizon is eluviation; their deposition in a given horizon is called illuviation.) The B horizon passes gradually into underlying material that’s relatively little affected by soil processes; it’s called the C horizon (but not universally considered to really be a soil horizon!).
The precise nature of the physicochemical processes that mobilize, transport, and deposit iron and, especially, aluminum are not yet entirely well understood. Iron, when weathered from parent minerals, is probably complexed with organic compounds and then precipitated as insoluble oxides lower down in the soil profile where the carrier organic compounds are degraded. The aluminum presents more of a problem. The aluminum is not actually put into true solution, but neither can it make its way down the profile as large clay-mineral particles. It probably travels either as a colloid (i.e., particles so fine as to be in the range between true solution and fine mineral particles) or physically as very fine, submicron-size clay-mineral particles.
Where the average water table lies far below, the B horizon tends to be diffuse; where the average water table is not far below, the B horizon tends to be more concentrated, and its colors stronger. (In areas where the average water table lies close to the surface, the B horizon tends instead to be gray from deposition of compounds containing iron in the reduced, ferrous state, as well as clay minerals.)
Now for something entirely different: a capsule description of a typical soil from the mid-continent of North America (Figure 2-29) Such soils are common in a wide area including western Kansas, western Nebraska, eastern Colorado, most of the Dakotas, and on into south-central Canada. In the traditional terminology, such soil would be called chernozem soils (from the Russian for “black soils”), chestnut soils, or brown soils. In such areas, with a semiarid (rainfall 15–25 inches) and strongly seasonal climate and dominantly grassland vegetation (now largely converted to agricultural use, especially in the eastern parts of the area), the balance between precipitation and evaporation is such that there is little net downward movement of water in the soil, with two important consequences: (1) the soil is largely a closed system, in the sense that chemical constituents produced by weathering remain in the soil rather than being carried away in solution in groundwater, and (2) the pH of soil waters tends to be alkaline rather than acidic. In such soils, upwards of a meter thick, the upper layer (A horizon) of the soil, beneath the surface organic layer, are a deep, homogeneous, and friable mixture of organic matter and mineral particles, not strongly leached, which is kept thoroughly mixed by the activity of burrowing organisms. This layer grades downward into a lighter-colored material with less organic matter, and then passes downward into a layer (the B horizon) in which calcium carbonate has been precipitated by mobilization of calcium ions in the upper layers and precipitation at deeper levels. The carbonate is precipitated as soil moisture is drawn back up toward the surface. In areas where the parent material contains carbonate minerals, the carbonated layer is better developed, but carbonate minerals tend to be deposited in the lower part of the soil profile even in the absence of carbonate minerals in the parent material. Why? Because of the common presence of calcium ions in parent silicate minerals, and the presence of carbon dioxide in the surface waters, to supply the carbonate ion.
2.9.8 Classification of Soils
Now, on to the troublesome and confusing matter of soil classification. Early classifications, before the middle of the 1900s, relied heavily upon terms and concepts developed in the late 1800s by Russian soil scientists, who were true pioneers in the scientific study of soils. These terms are common still, but in recent decades a systematic and very detailed classification system, developed and continually refined by soil scientists at the U.S. Department of Agriculture, has come into widespread use, despite its complexity and almost absurdly non- intuitive terminology. Also, other newer classifications are in use in other parts of the world.
(The “almost absurdly” in the preceding paragraph a value judgment, I know, but it’s a widely shared view. Just for laughs, I am appending, as Figure 2-31, from the thick tome on soil taxonomy published by the Soil Survey Staff of the Department of Agriculture, the official names of the “great groups”, which are the third stage in the taxonomic hierarchy. Each of the 185 great groups is in turn subdivided into subgroups. There are nearly a thousand subgroups! This classification is a marvel of rational and synthetic classification and terminology. Also in Figure 2-30 is a typical page from the same source, giving a description of some of the subgroups of one of the great groups. Another other interesting point about this classification is that, in contrast to any other classification I know of, in any field of science, they insist upon capitalizing every soil name: Durorthidic Xerorthents, rather than durorthidic xerorthents.)
I think that what would be most valuable for you at this point is to have soil classification stripped to its barest essentials. I will do that for you in two ways. First, Figure 2-31 gives the soil “orders” of the USDA classification system. Even this most boiled-down presentation needs a few words of explanation. A pedon (rhymes with “head on”) is the basic soil unit: the column of soil, with its various horizons, in a small representative area of the order of a square meter. The epipedon is just the surface horizon of the pedon; it’s usually rich in organic matter. Second, Figure 2-32, in three parts, gives (A) a simple classification of the earth’s climatic zones, and corresponding to that, (B) the major vegetation types and (C) the major soil groups. The typical New England soil, described in the preceding section, is a podzol developed in a climate with severe winters (!) and with spruce–fir forest. (Minor note: the climate of southern New England is gradational into the colder end of the “humid” box in Figure 2-32 and the box for gray brown podzolic soil in Figure 2-32. The native forests in southern New England are a mixture of conifers and deciduous hardwoods.)
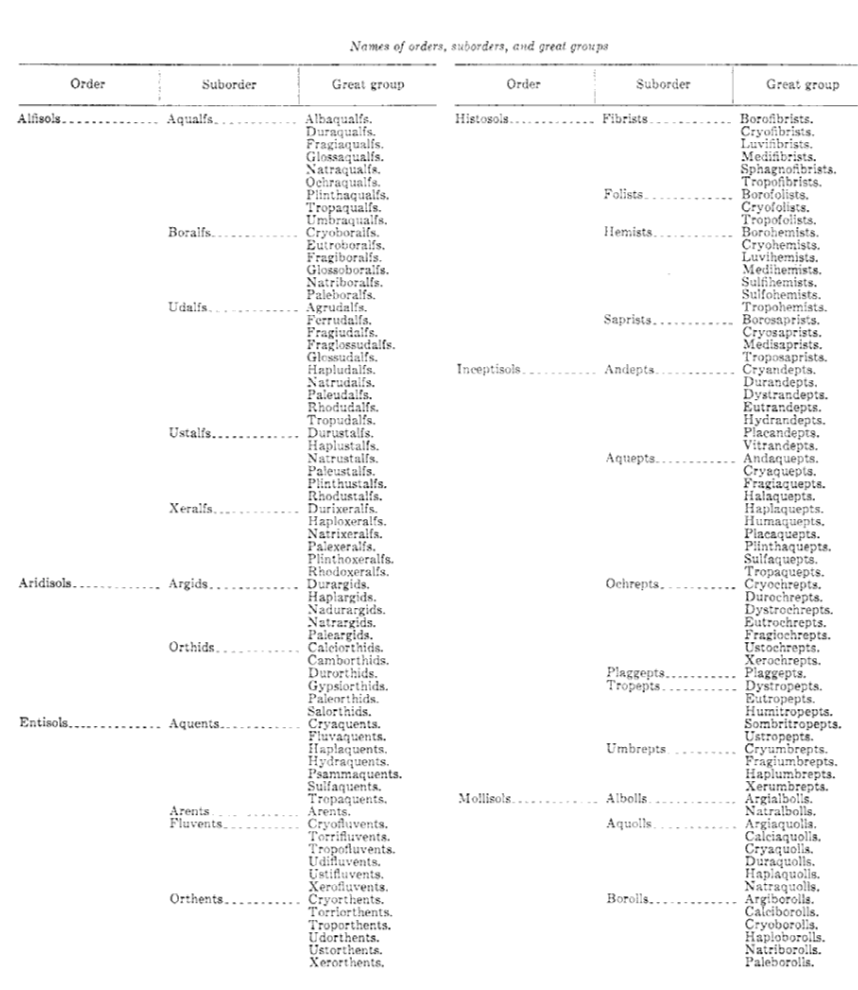
Figure 1-30. A) Orders, suborders, and great groups of the soil classification system developed by the Soil Survey Staff at the US Department of Agriculture. B). A random page from the system of soil taxonomy of the US Department of Agriculture. (From Soil Survey Staff, 1994)
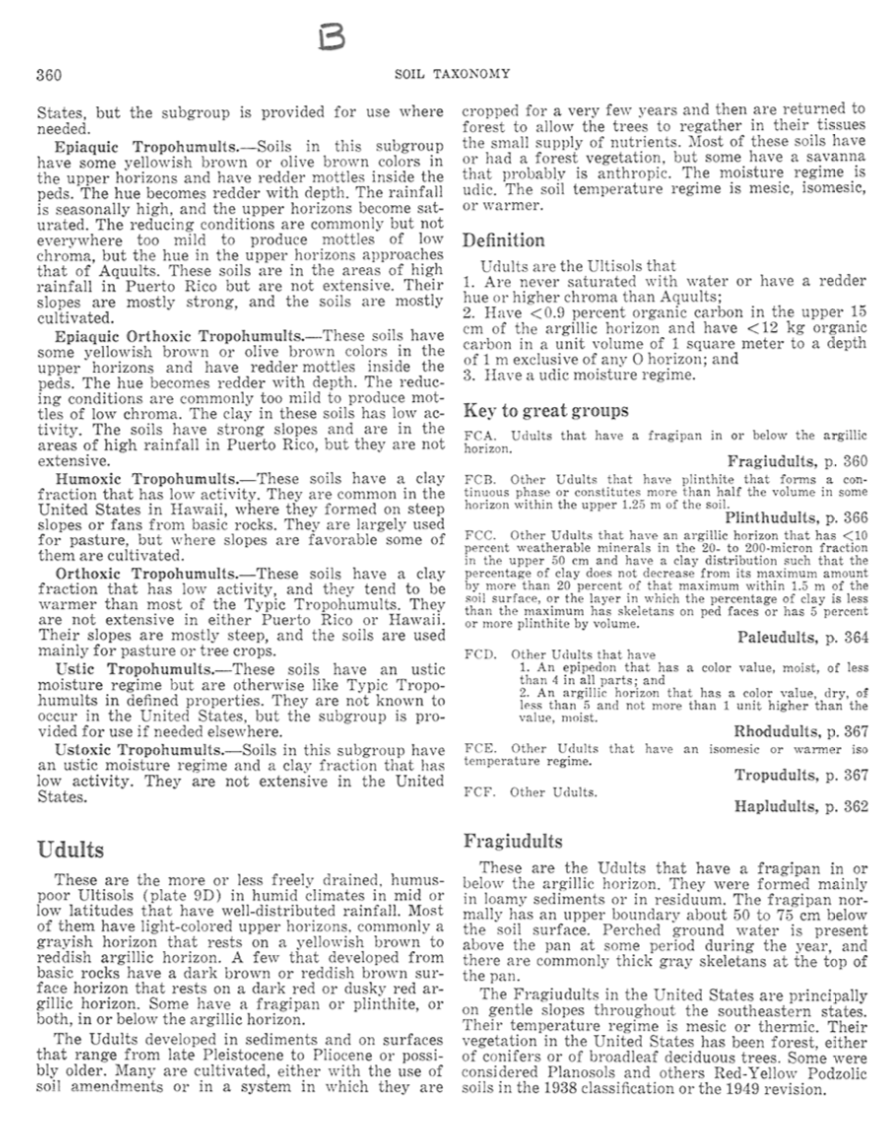
Figure 2-31. Orders of the US Department of Agriculture soil classification system. (Modified from Winegardner, 1996.)
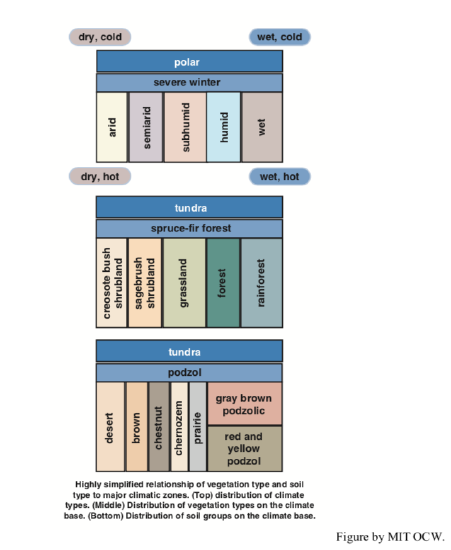
References
Brady, N.C. and Weil, R.R. 2002, The Nature and Property of Soils: Prentice Hall, 960 p.
FitzPatrick, E.A., 1986, An Introduction to Soil Science: Longman, 255 p.
Merrill, G.P., 1897, A Treatise on Rocks, Rock Weathering and Soils: Macmillan, 411 p.
Hunt, C.B., 1972, Geology of Soils; Their Evolution, Classification, and Uses: Freeman, 344 p.
Soil Survey Staff, 1994, Keys to Soil Taxonomy: US Department of Agriculture, Soil Conservation Service, 306 p.
Taylor, G., and Eggleton, R.A., 2001, Regolith Geology and Geomorphology: Wiley, 375 p.
Winegardner, D.L., 1996, An Introduction to Soils for Environmental Professionals: Lewis Publishers, 270 p.