8.1: Front Matter
- Page ID
- 14555
\( \newcommand{\vecs}[1]{\overset { \scriptstyle \rightharpoonup} {\mathbf{#1}} } \)
\( \newcommand{\vecd}[1]{\overset{-\!-\!\rightharpoonup}{\vphantom{a}\smash {#1}}} \)
\( \newcommand{\id}{\mathrm{id}}\) \( \newcommand{\Span}{\mathrm{span}}\)
( \newcommand{\kernel}{\mathrm{null}\,}\) \( \newcommand{\range}{\mathrm{range}\,}\)
\( \newcommand{\RealPart}{\mathrm{Re}}\) \( \newcommand{\ImaginaryPart}{\mathrm{Im}}\)
\( \newcommand{\Argument}{\mathrm{Arg}}\) \( \newcommand{\norm}[1]{\| #1 \|}\)
\( \newcommand{\inner}[2]{\langle #1, #2 \rangle}\)
\( \newcommand{\Span}{\mathrm{span}}\)
\( \newcommand{\id}{\mathrm{id}}\)
\( \newcommand{\Span}{\mathrm{span}}\)
\( \newcommand{\kernel}{\mathrm{null}\,}\)
\( \newcommand{\range}{\mathrm{range}\,}\)
\( \newcommand{\RealPart}{\mathrm{Re}}\)
\( \newcommand{\ImaginaryPart}{\mathrm{Im}}\)
\( \newcommand{\Argument}{\mathrm{Arg}}\)
\( \newcommand{\norm}[1]{\| #1 \|}\)
\( \newcommand{\inner}[2]{\langle #1, #2 \rangle}\)
\( \newcommand{\Span}{\mathrm{span}}\) \( \newcommand{\AA}{\unicode[.8,0]{x212B}}\)
\( \newcommand{\vectorA}[1]{\vec{#1}} % arrow\)
\( \newcommand{\vectorAt}[1]{\vec{\text{#1}}} % arrow\)
\( \newcommand{\vectorB}[1]{\overset { \scriptstyle \rightharpoonup} {\mathbf{#1}} } \)
\( \newcommand{\vectorC}[1]{\textbf{#1}} \)
\( \newcommand{\vectorD}[1]{\overrightarrow{#1}} \)
\( \newcommand{\vectorDt}[1]{\overrightarrow{\text{#1}}} \)
\( \newcommand{\vectE}[1]{\overset{-\!-\!\rightharpoonup}{\vphantom{a}\smash{\mathbf {#1}}}} \)
\( \newcommand{\vecs}[1]{\overset { \scriptstyle \rightharpoonup} {\mathbf{#1}} } \)
\( \newcommand{\vecd}[1]{\overset{-\!-\!\rightharpoonup}{\vphantom{a}\smash {#1}}} \)
\(\newcommand{\avec}{\mathbf a}\) \(\newcommand{\bvec}{\mathbf b}\) \(\newcommand{\cvec}{\mathbf c}\) \(\newcommand{\dvec}{\mathbf d}\) \(\newcommand{\dtil}{\widetilde{\mathbf d}}\) \(\newcommand{\evec}{\mathbf e}\) \(\newcommand{\fvec}{\mathbf f}\) \(\newcommand{\nvec}{\mathbf n}\) \(\newcommand{\pvec}{\mathbf p}\) \(\newcommand{\qvec}{\mathbf q}\) \(\newcommand{\svec}{\mathbf s}\) \(\newcommand{\tvec}{\mathbf t}\) \(\newcommand{\uvec}{\mathbf u}\) \(\newcommand{\vvec}{\mathbf v}\) \(\newcommand{\wvec}{\mathbf w}\) \(\newcommand{\xvec}{\mathbf x}\) \(\newcommand{\yvec}{\mathbf y}\) \(\newcommand{\zvec}{\mathbf z}\) \(\newcommand{\rvec}{\mathbf r}\) \(\newcommand{\mvec}{\mathbf m}\) \(\newcommand{\zerovec}{\mathbf 0}\) \(\newcommand{\onevec}{\mathbf 1}\) \(\newcommand{\real}{\mathbb R}\) \(\newcommand{\twovec}[2]{\left[\begin{array}{r}#1 \\ #2 \end{array}\right]}\) \(\newcommand{\ctwovec}[2]{\left[\begin{array}{c}#1 \\ #2 \end{array}\right]}\) \(\newcommand{\threevec}[3]{\left[\begin{array}{r}#1 \\ #2 \\ #3 \end{array}\right]}\) \(\newcommand{\cthreevec}[3]{\left[\begin{array}{c}#1 \\ #2 \\ #3 \end{array}\right]}\) \(\newcommand{\fourvec}[4]{\left[\begin{array}{r}#1 \\ #2 \\ #3 \\ #4 \end{array}\right]}\) \(\newcommand{\cfourvec}[4]{\left[\begin{array}{c}#1 \\ #2 \\ #3 \\ #4 \end{array}\right]}\) \(\newcommand{\fivevec}[5]{\left[\begin{array}{r}#1 \\ #2 \\ #3 \\ #4 \\ #5 \\ \end{array}\right]}\) \(\newcommand{\cfivevec}[5]{\left[\begin{array}{c}#1 \\ #2 \\ #3 \\ #4 \\ #5 \\ \end{array}\right]}\) \(\newcommand{\mattwo}[4]{\left[\begin{array}{rr}#1 \amp #2 \\ #3 \amp #4 \\ \end{array}\right]}\) \(\newcommand{\laspan}[1]{\text{Span}\{#1\}}\) \(\newcommand{\bcal}{\cal B}\) \(\newcommand{\ccal}{\cal C}\) \(\newcommand{\scal}{\cal S}\) \(\newcommand{\wcal}{\cal W}\) \(\newcommand{\ecal}{\cal E}\) \(\newcommand{\coords}[2]{\left\{#1\right\}_{#2}}\) \(\newcommand{\gray}[1]{\color{gray}{#1}}\) \(\newcommand{\lgray}[1]{\color{lightgray}{#1}}\) \(\newcommand{\rank}{\operatorname{rank}}\) \(\newcommand{\row}{\text{Row}}\) \(\newcommand{\col}{\text{Col}}\) \(\renewcommand{\row}{\text{Row}}\) \(\newcommand{\nul}{\text{Nul}}\) \(\newcommand{\var}{\text{Var}}\) \(\newcommand{\corr}{\text{corr}}\) \(\newcommand{\len}[1]{\left|#1\right|}\) \(\newcommand{\bbar}{\overline{\bvec}}\) \(\newcommand{\bhat}{\widehat{\bvec}}\) \(\newcommand{\bperp}{\bvec^\perp}\) \(\newcommand{\xhat}{\widehat{\xvec}}\) \(\newcommand{\vhat}{\widehat{\vvec}}\) \(\newcommand{\uhat}{\widehat{\uvec}}\) \(\newcommand{\what}{\widehat{\wvec}}\) \(\newcommand{\Sighat}{\widehat{\Sigma}}\) \(\newcommand{\lt}{<}\) \(\newcommand{\gt}{>}\) \(\newcommand{\amp}{&}\) \(\definecolor{fillinmathshade}{gray}{0.9}\)Living with Volcanoes
A volcano is any mountain or vent through which volcanic materials surface. This definition is broad to encompass all the potential types of volcanoes.
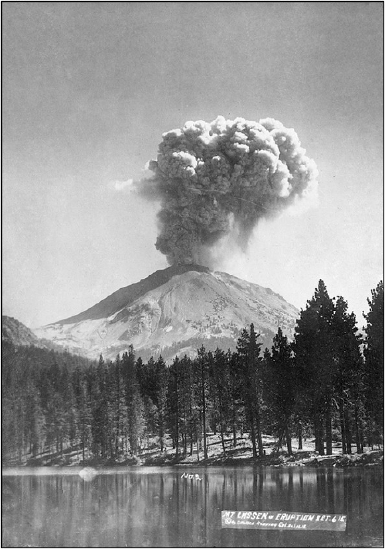
Would you be willing to live near a volcano? Some of us will say “NO WAY!”, while others may agree to a volcanic neighbor. Surprisingly, more than 500 million people live in areas at risk of a volcanic eruption. Some live on or near an active volcano, which has erupted in recent memory and may even be currently erupting (Figure 8.1; read more about the 1915 Lassen Peak eruption). Many more live near volcanoes that are considered dormant, experiencing a volcanically quiet period over the past 10,000 years but with the potential to erupt in the future. Extinct volcanoes are those that cannot erupt in the future, as their source of molten rock is no more.
Some of you may be thinking, “why would anyone live near an active volcano”?! Well, consider, why do you live in California? California is a geologically hazard-rich state: we have earthquakes, wildfires, landslides, volcanoes, tsunamis, sea-level rise, and much more. Why, then, do so many of us live here? It’s likely that you work, live, and go to school here. For many, family are nearby; others stay due to financial situations. Some love the access to beaches, mountains, and/or the desert. In general, you have weighed the pros and cons and have opted to remain here in the Golden State. Hazards scientists refer to this decision as acceptable risk: weighing the benefits of our location against the likelihood of its hazards impacting us.
In terms of volcanic risk, not all volcanoes erupt explosively. For example, the type of volcano that forms the Hawaiian Islands erupts effusively, with lava running down the sides (flanks) of the volcano (Figure 8.2). Hawaiian citizens are familiar with this style of eruption and are aware the volcano will not erupt explosively. However, these eruptions may still be destructive, as demonstrated by the 2018 eruption of Kilauea.
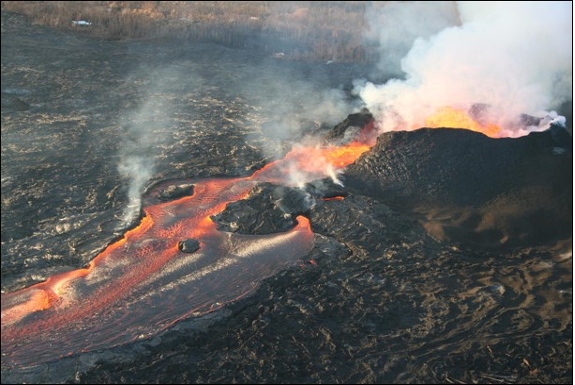
Explosive eruptions are more likely for the islands of Sumatra and Java or the volcanoes of the Pacific northwest (Figure 8.3). These volcanoes can be very dangerous, particularly for densely populated communities nearby. Because human settlements have been built around active and potentially explosive volcanoes, it is important to understand why these volcanoes are dangerous, while others, like those in Hawaii, carry less risk.
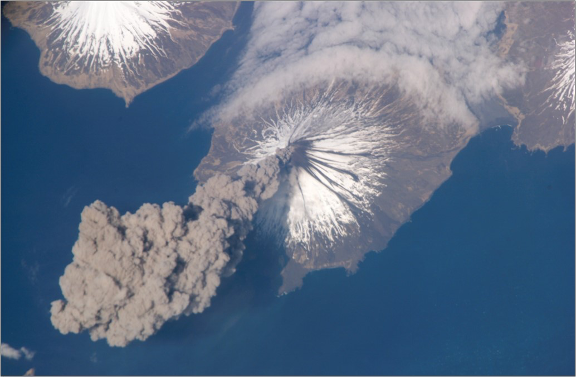
A person who studies volcanoes is referred to as a volcanologist. A volcanologist is a geoscientist who studies volcanic processes, styles, and eruptions. Like many other geoscientists, working with other disciplines is common, with a heavy influence from both math and technology. Many of these geoscientists are employed by universities where they teach and/or do research, and state and federal agencies, including geological surveys, like the California Geological Survey or United State Geological Survey (USGS). Additional career pathways are available in the private sector including in mining and natural resource extraction or in hazard mitigation and assessment. Many of these career options require a college degree and postgraduate work. If you are interested, talk to your geology instructor for advice. We recommend completing as many math and science courses as possible (chemistry is incredibly important for mineralogy). Also, visit National Parks, CA State Parks, museums, gem & mineral shows, or join a local rock and mineral club. Typically, natural history museums will have wonderful displays of rocks, including those from your local region. Here in California, there are a number of large collections, including the San Diego Natural History Museum, Natural History Museum of Los Angeles County, Santa Barbara Museum of Natural History, and Kimball Natural History Museum. Many colleges and universities also have their own collections/museums.
Melt Composition
Recall that igneous rock classification is in part based on the chemistry or mineral content of the sample. Ultramafic rocks are igneous rocks composed primarily of olivine and a lesser amount of calcium-rich plagioclase and pyroxene, whereas felsic rocks are composed primarily of quartz, muscovite and potassium feldspar. Igneous rocks are the crystallized result of a cooled melt, and the minerals that form during cooling depend on the chemical composition of the melt. For example, a mafic magma will form a mafic rock containing a large amount of the ferromagnesian minerals pyroxene and amphibole, but will not contain quartz (the mineral that is always present in felsic rocks). We classify a rock according to its chemistry; likewise, in order to understand the type of volcano produced by a specific magma, we need to consider its chemistry.
Partial Melt Composition
Recall that magma is generated by partial melting of a source rock, and if we refer to Bowen’s Reaction Series (Figure 8.4), we notice that minerals have different melting temperatures. If a rock undergoes partial melting, the minerals that melt are those minerals on the bottom of the Reaction Series that are rich in silica (SiO2) and poor in iron and magnesium. This means that magma generated by partial melting will be richer in silica and contain less iron and magnesium than the source rock (the rock that partially melted). The different magma types (and the solid rock forms that they make) are listed in order of decreasing iron and magnesium, and increasing silica, with ultramafic rocks as the most iron and magnesium rich and silica poor, and felsic rocks as the most deficient in iron and magnesium but containing the most silica. Therefore, partial melting of a mantle rock that has an ultramafic composition will produce a mafic magma; likewise, partial melting of a mafic rock can produce an intermediate magma type, and partial melting of an intermediate rock can produce felsic magma.
There are other ways that felsic and intermediate magmas are produced; for example, magma that is moving upward (ascending) through the crust may melt and incorporate some of those crustal rocks, changing the magma’s composition. Recall that the continental crust is the lowest density rock type of the Earth, of granitic composition, and quite thick at convergent plate boundaries; at convergent plate boundaries, magma may have to travel through this thick portion of the continental crust. At any time before the magma is completely crystallized, it can melt the surrounding crust and end up with a more silica rich composition. That means an intermediate or even a mafic magma will become more felsic as it travels through the continental crust.
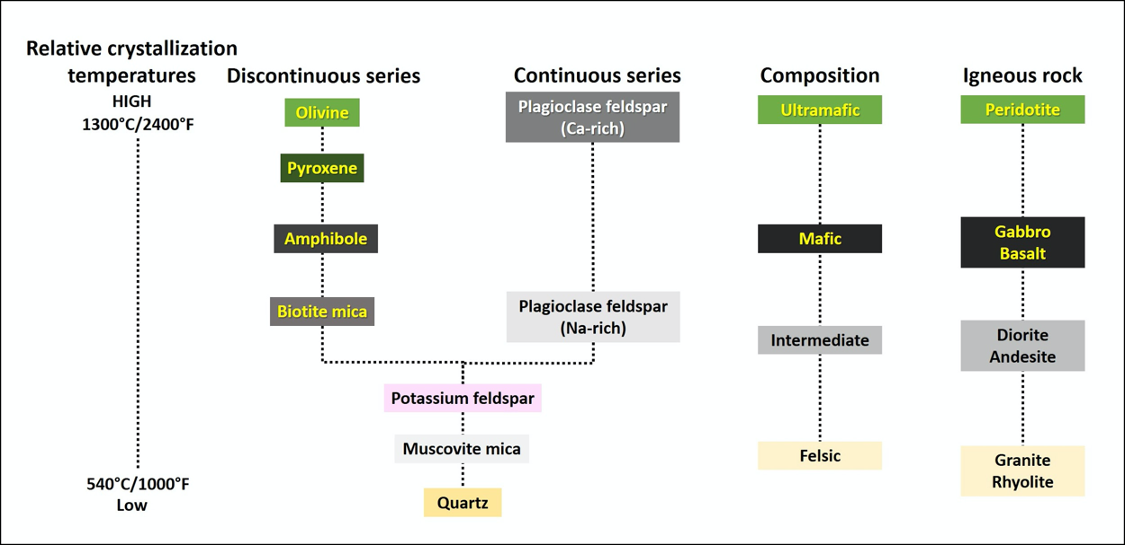
While most magma crystallizes below ground, a portion of it does erupt onto the surface of the Earth, either as a passive lava flow or under more explosive conditions such as the May 1980 eruption of Mount St. Helens, WA. Whether the style of eruption is passive or active (explosive) depends on the magma composition.
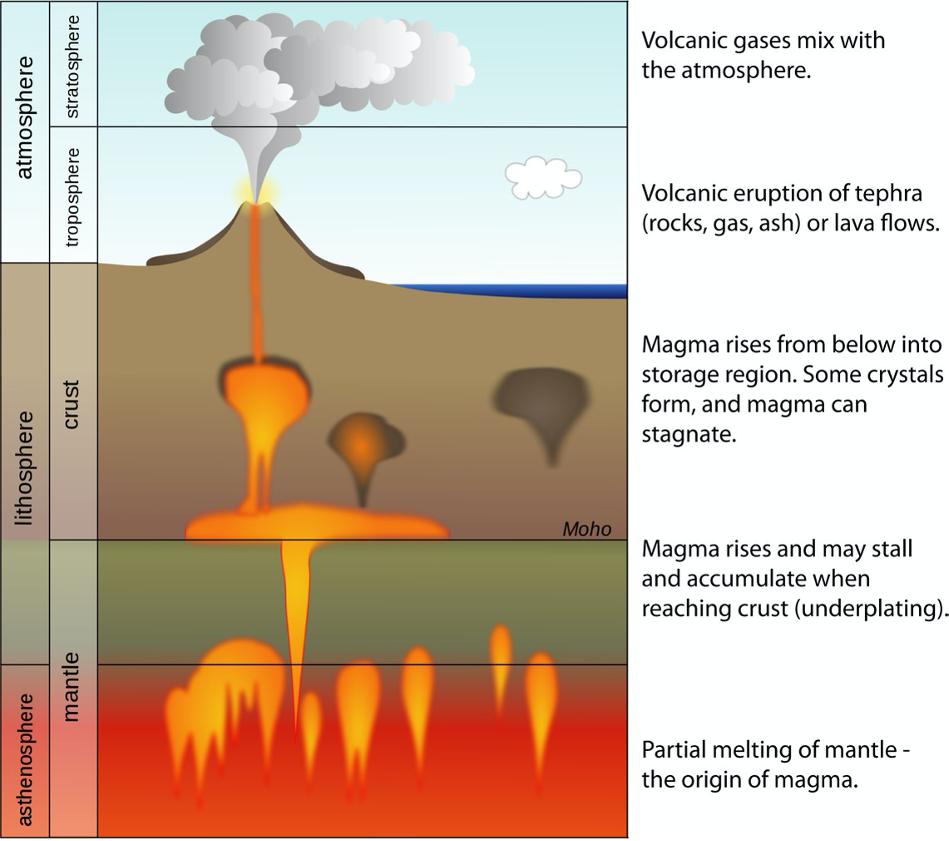
Viscosity
Most of the minerals in igneous rocks are silicate minerals, and all the minerals shown in Bowen’s Reaction Series belong to the silicate mineral group. All silicate minerals have crystal structures containing silica tetrahedra. These silica tetrahedra can be linked in a variety of ways, to form sheets, linked chains, or a 3-dimensional framework. The lower temperature minerals (quartz, muscovite and potassium feldspar) have more linked tetrahedra than the high temperature minerals (olivine, amphibole and pyroxene). Recall from our mineral unit how silica tetrahedra are linked to form the mineral quartz. What do you think will happen to the silica tetrahedra when quartz melts? The crystal structure is lost, the regularly repeated structure is gone, but the tetrahedral links are maintained (though distorted). The bonds that link these tetrahedra are strong, and magma temperatures are not high enough to break every bond. This means that felsic magmas in which quartz crystallizes will have a lot of these linked tetrahedra, whereas mafic magmas, which do not contain enough silica to crystallize quartz, will contain fewer linked tetrahedra. Why is the silica content, and specifically the amount of linked tetrahedra, so important? A large amount of linked silica tetrahedra will result in a magma or lava that is very viscous: it will not flow easily. Viscosity is a material's resistance to flow and is affected by the chemistry, temperature, and gas content of the melt.
Imagine sitting down to a breakfast (or brunch) of pancakes with syrup. Where do you store your syrup? In the refrigerator or pantry? Syrup stored in the refrigerator is cold and therefore pours slowly, whereas syrup stored in the pantry flows more easily. Consider what happens to the syrup when you heat it in the microwave; it will now flow even more readily. The syrup’s viscosity, and the connections between the sugar crystals in the syrup, is affected by the temperature. Cold syrup has a higher viscosity, while hotter syrup has a lower viscosity. This is also true for molten rock: in general, melts that are hotter tend to flow more easily. These runny lavas also typically contain fewer linked silica tetrahedra, and readily allow gases to escape. Mafic lavas, sourced from the hotter mantle or oceanic crust and containing fewer silica chains, will flow faster than intermediate or felsic lavas. The viscosity difference between differently composed magmas affects the overall shape or appearance of the volcano, as well as the style of eruption (effusive vs. explosive).
Composition | Temperature | Gas content | Viscosity | Explosivity potential |
---|---|---|---|---|
Mafic Olivine, pyroxene, amphibole |
High | Gases readily escape | Low (runny) | Low |
Intermediate Amphibole, biotite, Na-rich plagioclase feldspar |
Moderate | Gases become somewhat trapped | Moderate | Moderate |
Felsic Quartz, muscovite, potassium feldspar |
Low | Gases are trapped | High (sticky) | High |
Volcanic Materials
Lava Flows
Mafic lavas include pillow basalts, pahoehoe, and a’a lavas. Pillow basalts are the result of an underwater eruption. These pillow-shaped bodies, commonly erupted at mid-ocean ridges and oceanic hot-spots, form as a result of rapid cooling (Figure 8.6). The exterior of the pillow is commonly a volcanic glass, while the interior may be crystalline.
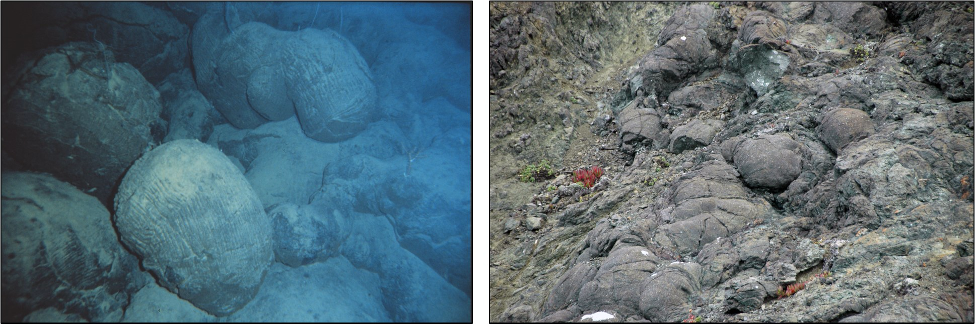
Pahoehoe lava flows have a very low viscosity and are typically runny with a smooth and lobate shape. When cooled, they form rope-like patterns (Figure 8.7). Due to their runny nature they are capable of moving great distances from their volcanic vent.
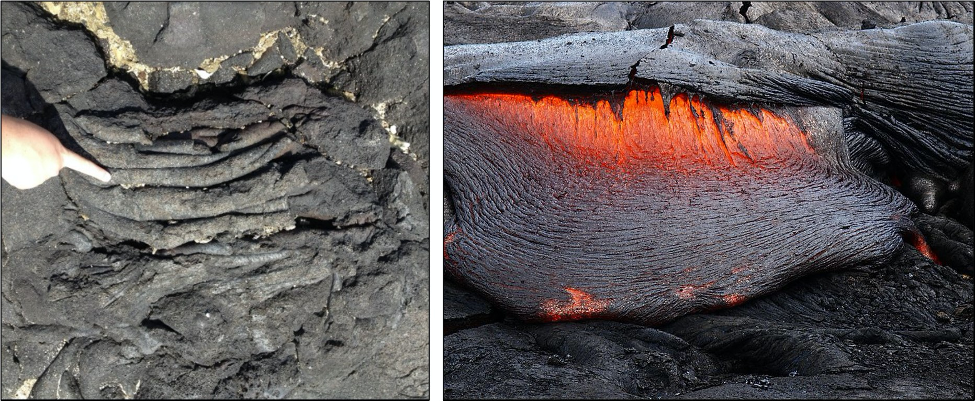
A’a lava flows run slightly cooler and therefore are a bit more viscous. This causes the lava surface to appear uneven and broken. When cooled, the rock remains jagged and rough (Figure 8.8). If you could choose to hike over a cooled pahoehoe or an a’a flow, the pahoehoe flow would be much kinder on your feet and ankles (and hiking boots!).
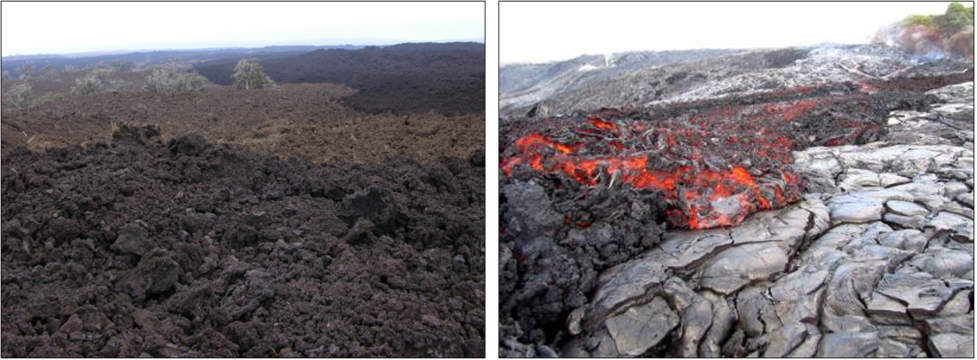
Intermediate to felsic lavas are typically referred to as block lavas or rhyolitic or dacite lavas. These lava flows are very viscous and are unable to move very far from their vent. These lavas can pile up on themselves and produce a mound or dome of lava (Figure 8.9).
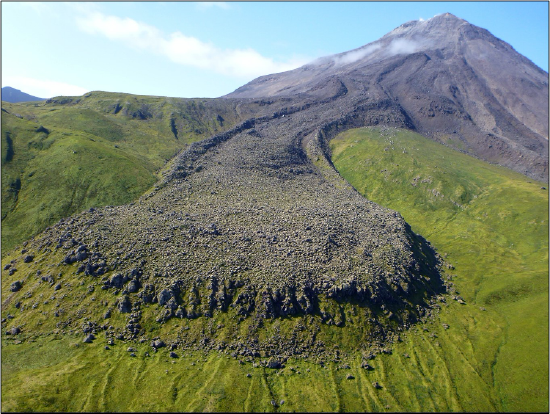
Columnar Joints form when lava flows cool, solidify, and contract. Long vertical cracks, or joints, form within the brittle rock to allow for the shrinkage. Viewed from above, the joints form polygons with 5, 6, or 7- sides, and angles of approximately 120° between sides (Figure 8.10). Devil’s Postpile National Monument here in California has amazing examples of these columnar joints. Watch this video for a virtual field trip to Devil’s Postpile.
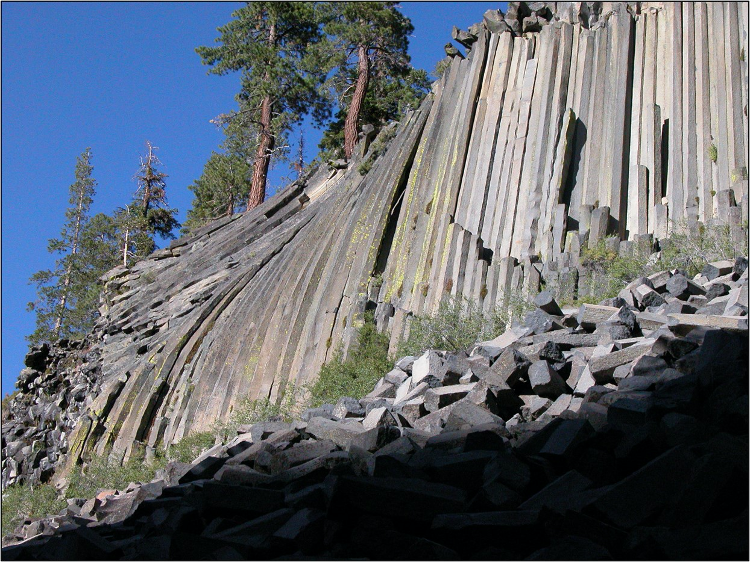
Volcanic Gases
Magmas contain dissolved gases that expand as the magma migrates up through the crust. As the gases expand, bubbles form, much like the bubbles produced after you crack open a soda. These gases become an important driving factor behind eruptions. If the gas bubbles are able to be vented from the magma slowly, the eruption will be effusive, or gentle. Effusive eruptions typically produce lower viscosity lava flows and/or lava fountains, where the height of the lava fountain depends on the gas content of the lava. If the gas bubbles become trapped in the magma and cannot be vented to the surface, however, pressure begins to build. Eventually the pressure exceeds the strength of the rock, and the resulting eruption can be explosive and violent. Explosive eruptions are typical of more viscous magmas.
Common volcanic gases include water vapor, carbon dioxide, and sulfur dioxide, but, depending on the chemistry of the magma and host rock, other gases are possible. Certain volcanic gases can pose significant hazards to local populations, causing problems for health, crops, and to infrastructure. For more information about gases impacting a broader community, read this USGS Fact Sheet: Invisible CO2 Gas Killing Trees at Mammoth Mountain, California or explore the 1986 disaster at Lake Nyos, Cameroon in Africa.
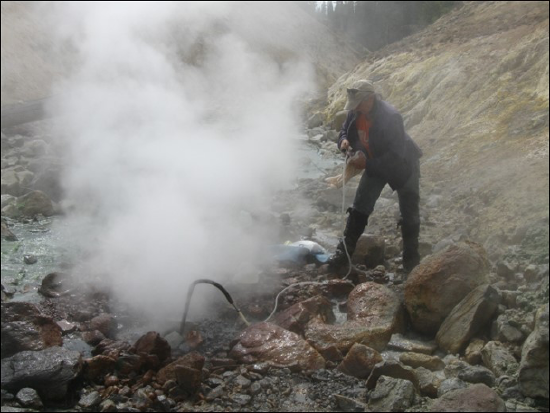
During the Precambrian, volcanic gases were a likely source of our early atmosphere and oceans. Gases emitted by volcanoes continue to influence the atmosphere, for example the Mt. Pinatubo eruption in 1991 released large quantities of sulfur dioxide that resulted in global cooling by 1°F. While this may not seem like a large number, a single degree globally can have serious ramifications on weather at a local level. While some volcanic eruptions have had a global impact on the climate, the total amount of volcanic outgassing during human history does not come close to measuring up to how rapidly humans are altering the composition of the atmosphere.
Pyroclastic Debris
Pyroclastic debris, tephra, or cinders are the fragmented volcanic material released during a volcanic eruption. Smaller sized material can travel great distances along air currents. Pyroclastic debris are classified according to their size.
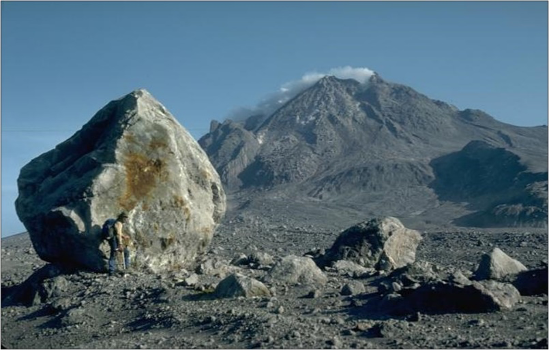
Larger pieces (>2.5 in or 6 cm in diameter) are referred to as volcanic blocks and bombs. Volcanic blocks are typically jagged shaped pieces dislodged from the volcano during an eruption (Figure 8.12).
Volcanic bombs are semi-molten or molten blebs of lava that get hurled through the air during an eruption. As they fly through the air, they begin to cool and harden. Smaller pieces can be completely solidified by the time they impact the ground, while larger pieces may still be partially molten upon impact and flatten on one side (Figure 8.13). Both volcanic blocks and bombs typically remain closer to their volcanic source, although if an eruption is very gas-rich and therefore explosive, they can be hurled miles away.
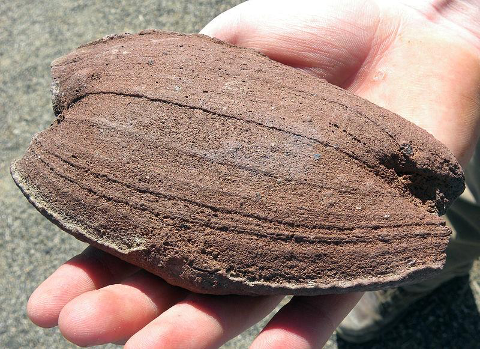
Lapilli, or cinders, are golf-ball to pea-sized vesicular or glassy pieces (Figure 8.14). These pieces can be carried upward within a volcanic plume and downwind in a volcanic cloud, but fall to the ground as the eruption cloud cools. These pieces can quickly pile up on roofs of structures, which can result in cave-ins.
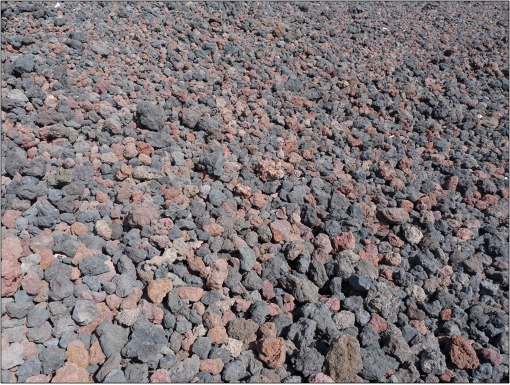
Volcanic ash is the smallest of the fragmented material. Volcanic ash is different from the ash of a fire. Wildfires, bonfires, or fireplace ash is created as a result of a chemical reaction with organic material. Volcanic ash is rock or glass particles generated during an explosive eruption (Figure 8.15). Volcanic ash can be so small it can pass through the weave of fabric, even denim. After an explosive eruption you may see people with bandanas or t-shirts covering their mouth and nose. The ash may be small enough to pass through this fabric, so, typically, medical grade masks (N-95 or better) are the best barrier for keeping the particulates out of your respiratory system. We will return to volcanic hazards in a later section.
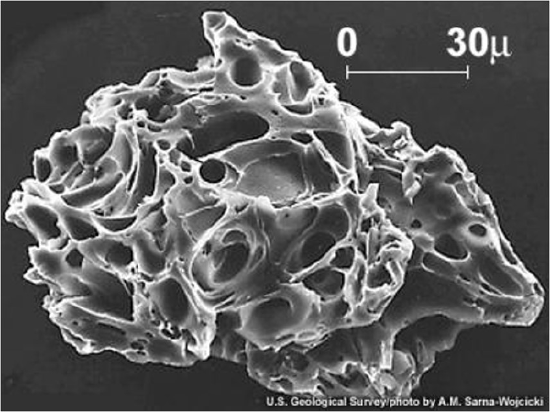
Volcano Types
The size, shape, and eruptive style of any volcano ultimately depends on the magma composition and types of materials erupted. Mafic lava flows can travel quite far before solidifying completely. These lava flows can even form lava tubes, through which molten lava flows like a river under a cave-like crust of hardened lava rock. A shield volcano typically forms from mafic lava flows. Shield volcanoes are very broad at their base and have relatively gentle slopes. In map-view they look similar to Captain America’s shield, hence their name. Shield volcanoes are the largest of the volcano types, although they are gentle giants. One of the largest landforms and biggest volcano in our solar system is a shield volcano on Mars, Olympus Mons, which is roughly the size of Arizona (Figure 8.16). Shield volcanoes are frequently associated with mantle plumes, although some form at divergent boundaries, either on land or on the seafloor.
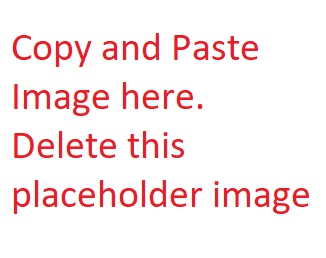
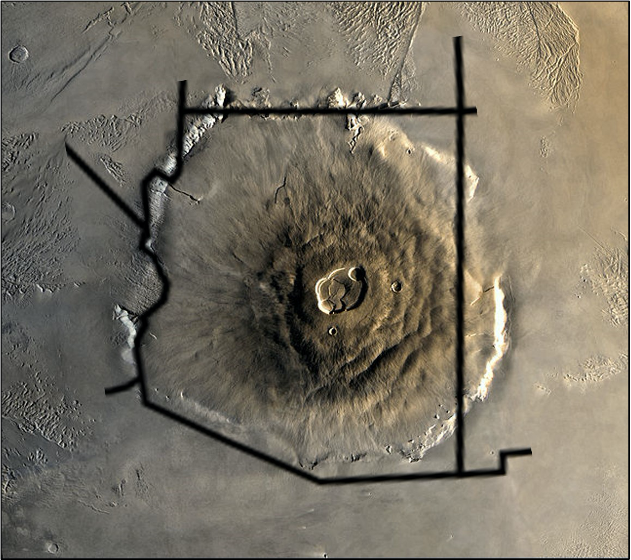
There are several locations around the world where large amounts of mafic basalt flows are found, without having formed a shield volcano. These places include the Deccan Traps in India, Siberian Traps in Russia, Central Atlantic Magmatic Province (CAMP), and the Columbia River Basalt Group of the US Pacific northwest. Such vast outpourings of mafic lava are called flood basalts or large igneous provinces (LIPs) and are believed to be caused by mantle plumes (hotspots) that partially melt the mantle beneath the Earth’s crust. The mafic magma generated by such a mantle plume reaches Earth’s surface through many fractures or fissures instead of one central vent and can output millions of square kilometers of lava over hundreds of thousands to millions of years.
A cinder cone (spatter cone) forms from cooler mafic lava released through a vent. The cooler lava traps gases, increasing the explosivity of the volcano and generating pyroclastic debris or cinders. The debris piles up around the vent, forming a small, conical shaped volcano (Figure 8.17). Typically, cinder cones are the smallest of the volcano styles. Most are made up of fragments of vesicular mafic rock (pumice or scoria). In many cases, these later became the sites of effusive lava flows after the gases have been vented from the magma. Most cinder cones are monogenetic, meaning that they formed during a single eruptive phase, lasting weeks to months. These cones are made almost entirely of loose fragments that have very little cohesion, allowing most to be quickly eroded away.
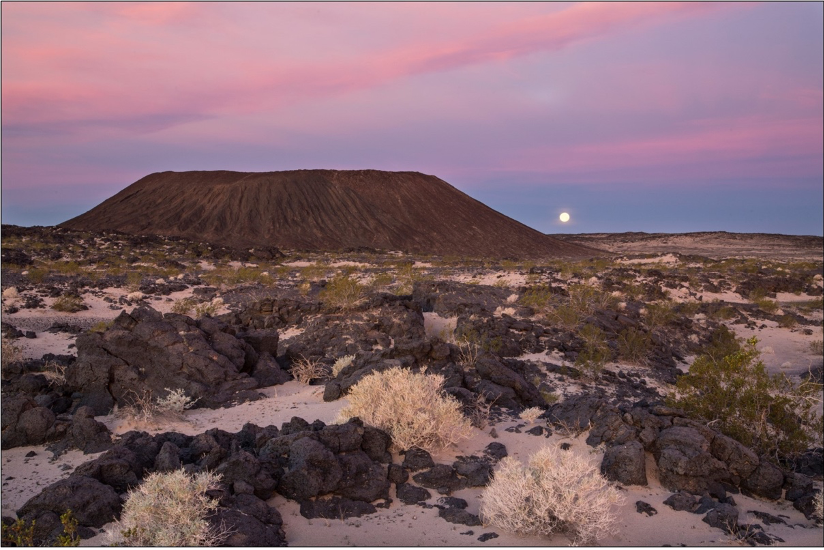
A composite volcano (or stratovolcano) forms from intermediate to felsic magmas erupting through a central vent. This volcano type gets its name because it is a composite of lava flows and pyroclastic materials produced during explosive eruptions (Figure 8.18). Recall that it is common to have dissolved gases within magma and that some gases may escape from the magma while still underground; however, the most spectacular release of gases occurs during an explosive eruption. At many such volcanoes, magma is stored in a magma chamber in the upper part of the crust.
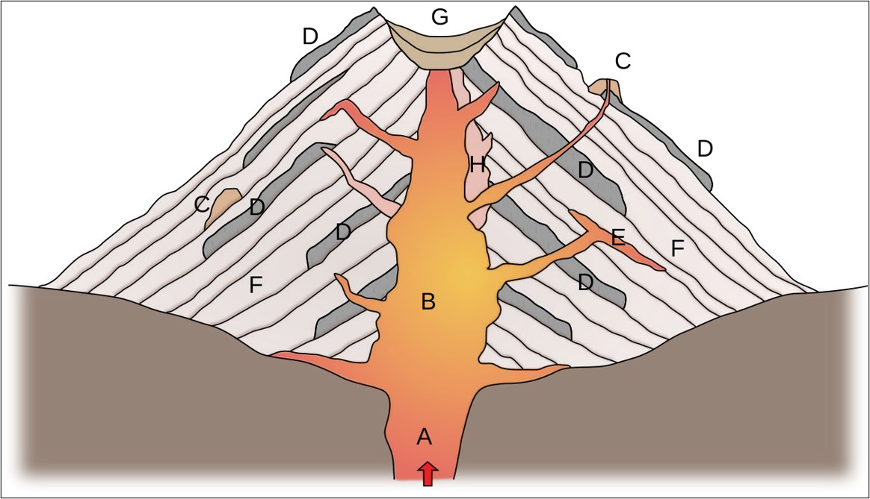
A lava dome is formed from viscous block lava that is resistant to flow, like thick toothpaste being squeezed from a tube. This lava plugs the volcanic vent, building up intense pressure; when the pressure exceeds the strength of the rock, the volcano rips apart in a violent eruption. Typically, spines grow out of lava domes and can indicate an upcoming eruption. Lava domes can exist on their own (Lassen Peak) or be associated with composite volcanoes (inside the crater of Mt. St. Helens). As a dome ages, its lavas evolve, becoming more silica rich and with a higher viscosity.
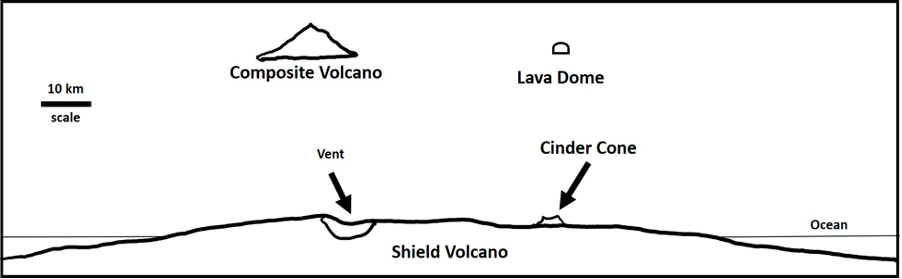
Volcano style | Size and shape | Eruption material | Composition | Viscosity & Explosivity | Common Tectonic Setting | Examples |
---|---|---|---|---|---|---|
Caldera |
Broad depression with flat bottom and steep sides. Generally >1 mi in diameter |
Mix; pyroclastics, lava flows |
Intermediate to felsic (eruption style) Mafic (subsidence style) |
High (eruption) Low to intermediate (subsidence) |
Subduction zones, continental hot spots (eruption), divergent boundaries, oceanic hot spot (subsidence) |
Long Valley, CA Yellowstone, WY (eruption) Kilauea, HI Bardarbunga, Iceland (subsidence) |
Shield | Large, broad shape (up to 125 mi across); not steep (slopes 2-10°) | Lava flows | Mafic | Low | Divergent boundaries; oceanic hot spots | Hawaiian Islands Iceland |
Cinder (scoria) cone | Small size (30 to 350 ft); steep (slopes >20°) | Pyroclastic debris | Mafic | Low to intermediate | Varies; common on the flanks of other volcanoes | Cinder Cone, CA Amboy Crater, CA |
Composite cone (strato- volcano) |
Medium size (1000’s of ft high); moderate steepness (10-30°) | Mix; pyroclastics, lava flows | Intermediate to felsic | Intermediate to high | Subduction zones |
Mt. Shasta, CA Mt. St. Helens, WA Mt. Fuji, Japan |
Lava dome (rhyolite dome) | Small size | Block lavas | Felsic | High | Subduction zones, continental hot spots |
Lassen Peak, CA Panum Crater, CA Sutter Buttes, CA |
Hot spots are volcanically active areas on the Earth’s surface that are likely a result of a mantle plume that rises from deep in the mantle toward the surface, resulting in melted rocks and volcanoes. These mantle plumes originate deep within Earth and are unaffected by the movement of the continents or the crust under the ocean. Mantle plumes appear to be stationary through time, but as the tectonic plate moves over the hot spot, a series of volcanoes are produced. There are a number of hot spots in the US, including under Hawaii and Yellowstone. Here in California, we have a hot spot situated beneath the Mammoth/Mono Lake region, an area home to Long Valley Caldera, which is heavily monitored by the California Volcano Observatory (CalVO).
The Yellowstone Hotspot: A hot spot beneath the North American plate is responsible for much of the geology in Yellowstone National Park in Wyoming. This park does not contain the typical volcanic features, as there is no conical shaped volcano. Instead much of the park sits within a series of three massive calderas; the largest is more than 30 miles across! During the last 2 million years, this hot spot has experienced three violent and explosive eruptions, with minor, less explosive eruptions in between. Partial melting of the overlying continental crust occurs as magma pools on the underside. The resulting magma has a high silica and gas content, which produces explosive eruptions like those identified by thick pyroclastic deposits in the geologic record. Want to learn more? Visit the Yellowstone NPS page.
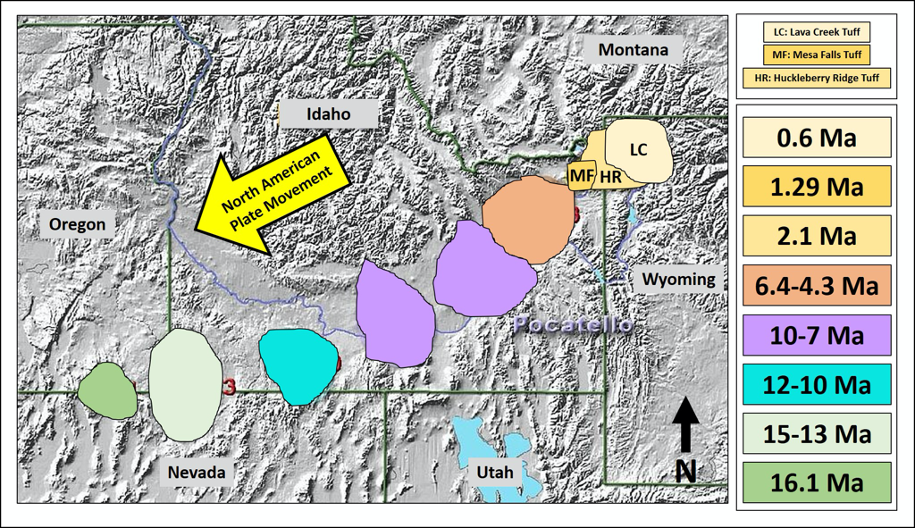
Eruption Styles
There are four types of eruptions with properties determined mostly by the silica and gas contents of magma. In order of increasing explosiveness, these are Hawaiian, Strombolian, Vulcanian, and Plinian eruptions. Any composition of magma can have an explosive eruption if the magma suddenly encounters water. Hot magma contacting groundwater or seawater causes the water to flash to steam. Explosive eruptions driven by water are called hydrovolcanic (or phreatic) eruptions.
Hawaiian eruptions are named after the characteristic eruptions of the volcanoes of the Hawaiian Islands. Hawaiian eruptions are effusive (flowing) rather than explosive because they erupt low-viscosity mafic lava. Hawaiian eruptions form shield volcanoes and can also take the form of fissure eruptions. Fissure eruptions occur when lava erupts from long cracks in the ground rather than from a central vent.
Strombolian eruptions, named for Mt. Stromboli in Italy, occur when mafic lava has higher viscosity and higher gas content. The sticky lava is ejected in loud, violent, but short-lived spattery eruptions. Clumps of gas-rich lava are thrown tens to hundreds of feet in the air and accumulate as scoria in a pile around the vent, forming cinder cones.
Vulcanian eruptions get their name from the volcanic Italian island of Vulcano, which itself takes the name of the Roman god of fire, Vulcan. In Roman mythology, Vulcan was the maker of armor and weaponry for the gods, and volcanic eruptions were attributed to him working in his forge. Vulcanian eruptions are far more explosive than Strombolian eruptions, and can blast tephra and gas to a height of 3 to 6 miles. The explosiveness is related to a build-up of pressure as the higher viscosity of intermediate silica content lava restricts the escape of gas. Vulcanian eruptions produce large quantities of ash, in addition to blocks and bombs.
Plinian eruptions are explosive eruptions of intermediate to felsic lava and can form eruptive columns up to 30 miles high. The origin of the name is the eruption of Vesuvius in 79 CE, which buried the towns of Pompeii and Herculaneum. The Roman admiral Gaius Plinius Secundus, also known as Pliny the Elder, attempted a rescue mission when he saw the column of ash and debris above Vesuvius, but died of unknown causes before he was able to reach Herculaneum.
Hydrovolcanic (phreatic) eruptions can be far more explosive than Plinian eruptions. They occur when water - in the form of groundwater, seawater, or even melting glacial ice or snow - comes into contact with magma. Heat from the magma abruptly changes water to steam, which can expand to more than a thousand times the original volume of water. This sudden expansion results in an explosive force that can blast a volcano to pieces and create large amounts of volcanic ash.
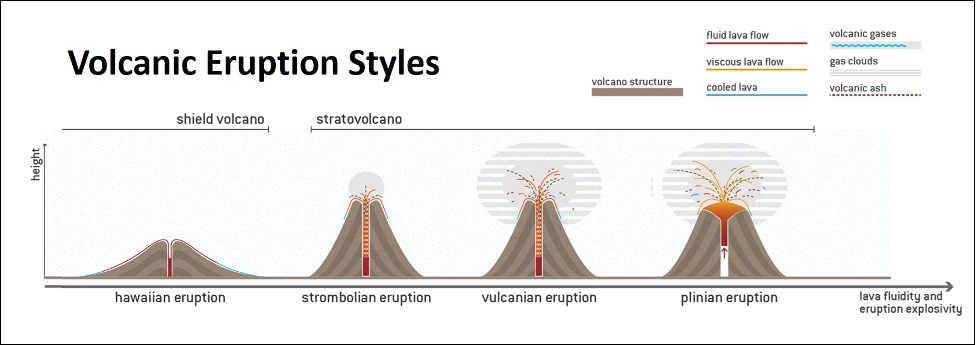
Volcanic Explosivity Index (VEI)
To determine the explosivity value of an eruption, geologists will calculate the volume of eruption products, measure the height of the eruption column, and make qualitative observations about the eruption impact.
This scale is logarithmic, with each interval on the scale representing a tenfold increase in observed ejecta criteria (except between VEI 0, VEI 1 and VEI 2). A value of 0 is given for non-explosive eruptions, defined as less than 10,000 m3 (350,000 cu ft) of tephra ejected. The largest volcanic eruptions in history, supereruptions, are given a magnitude of 8, representing a mega-colossal explosive eruption that can eject more than 1000 km3 (>240 cubic miles) of tephra and have a cloud column height of over 20 km (12 mi).
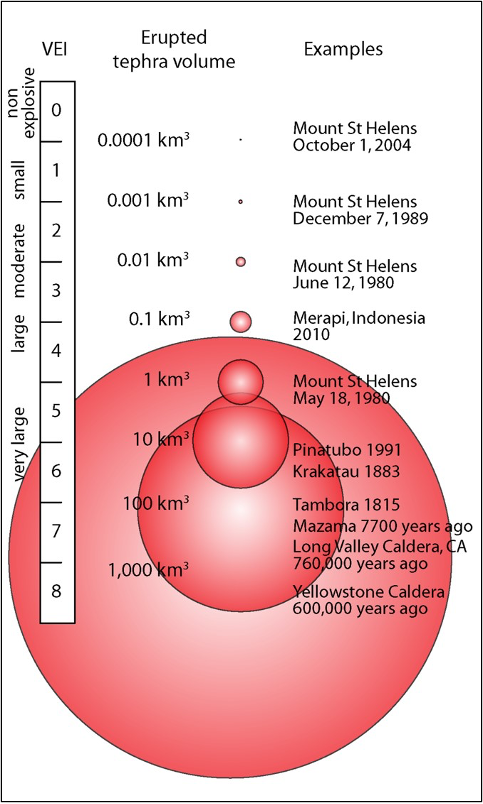
Volcanic Hazards
While shield volcanoes are more massive, they are far less dangerous to a population than the smaller composite volcanoes. Shield volcanoes produce runny lavas that may fountain at a vent, but usually end up flowing passively down the flanks of the volcano. While property damage may be significant (see the 2018 Kilauea eruption), anyone living on or near a shield volcano is not likely to perish due to the lava flows. This is not the case for the explosive eruptions of composite volcanoes that are associated with many distinct volcanic hazards (Figure 8.22). These eruptions produce a lot of pyroclastic debris, that range in size from microscopic ash to huge volcanic blocks. These pyroclastic debris first travel high up into the atmosphere as an eruption column, which spreads outward with the prevailing wind direction. As the eruption widens the central vent, the eruption column decreases its upward momentum. As a result, the pyroclastic material collapses down the flanks of the volcano as a pyroclastic flow: a very dangerous, fast moving, mixture of hot volcanic material and noxious gases.
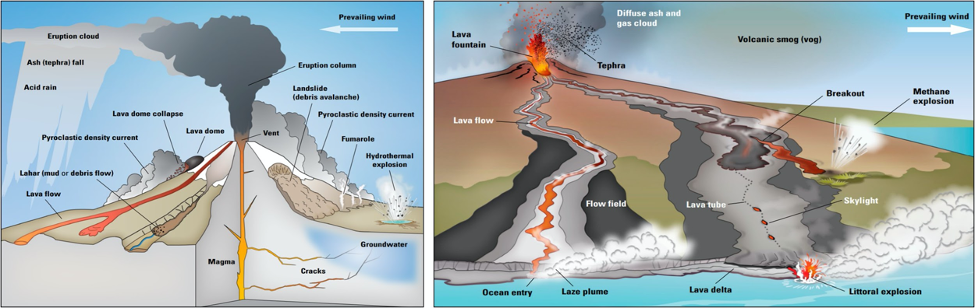
Many composite volcanoes are capped by snow and ice, and even a small eruption can result in meltwater running down the sides of the volcano, which creates volcanic mudflows or lahars. The meltwater easily erodes the ash and other volcanic debris on the flanks of the volcano and results in a fast-moving slurry of mud and larger material such as trees and boulders. Lahars move swiftly through river channels and can endanger any town or city that is built in the low-lying areas downstream from the volcano. Lahars can also be generated by large amounts of rainfall in the area. In general, lahars are uncommon in the Lassen Peak region of northern California; however, there are a few deposits found in drainages, demonstrating that these valleys are susceptible to future lahars. In the Cascade region of Washington and Oregon, there are several ancient volcanic mudflow deposits recognized. Many of the communities in the Pacific northwest have lahar monitoring systems available to detect these mudflows.
Volcanoes can be monitored in a variety of ways, including via seismic activity (earthquakes), changes in slope, gas emissions, thermal imagery, and more (Figure 8.23). By combining information from these sources, along with careful observations made on the ground and from the air, and a thorough knowledge of how volcanoes work, geologists can get a good idea of the potential for a volcano to erupt in the near future (months to weeks, but not days). They can then make recommendations to authorities about the need for evacuations and restricting transportation corridors.
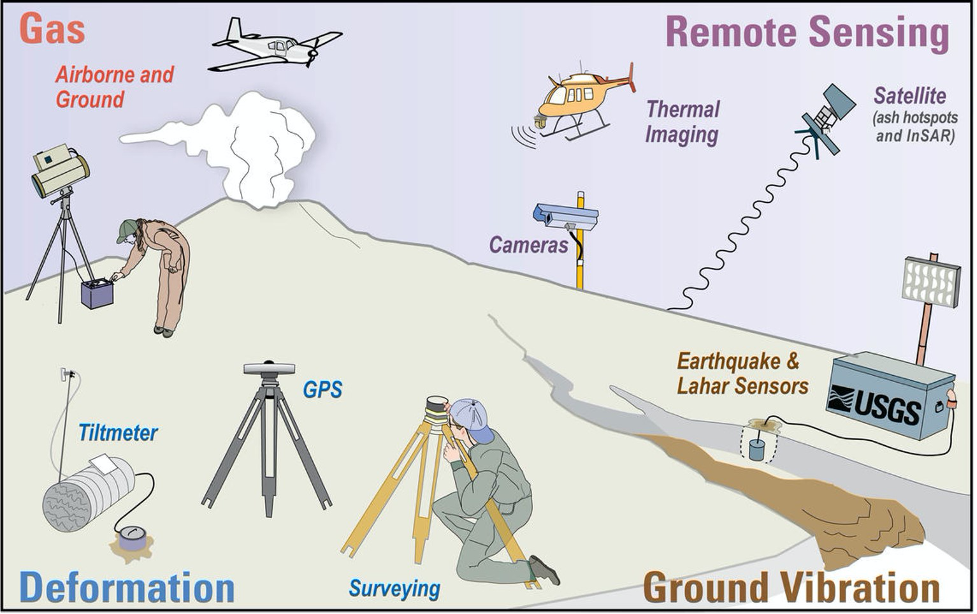
Attributions
-
Figure 8.1: “Eruption from Manzanita Lake” (Public Domain; USGS)
-
Figure 8.2: “Kilauea Volcano – Fissure 8 Aerial” (Public Domain; USGS)
-
Figure 8.3: “Eruption of Cleveland Volcano, Aleutian Islands, Alaska” (Public Domain; NASA)
-
Figure 8.4: “Bowen’s Reaction Series and Associated Igneous Rocks” (CC-BY 4.0; Chloe Branciforte, own work)
-
Figure 8.5: “Basic Process of Magma Formation” (Public Domain; based on an image from Woudloper via USGS)
-
Table 8.1: “Igneous Compositions” (CC-BY 4.0; Chloe Branciforte, own work)
-
Figure 8.6: Derivative of {“Pillow Lavas Off Hawaii” (Public Domain, NOAA via Wikimedia Commons and “Pillow Basalts” (CC-BY 2.0; James St. John via Flickr)} by Chloe Branciforte
-
Figure 8.7: Derivative of {“Pahoehoe” (CC-BY 4.0, Chloe Branciforte, own work) and “Pahoehoe Lava Forming Ropy Lava” (CC-BY-SA 4.0; Rufiyaa via Wikimedia Commons)} by Chloe Branciforte
-
Figure 8.8: Derivative of {“A’a Flow” (CC-BY 4.0, Chloe Branciforte, own work) and “A’a” (Public Domain; USGS HVO)} by Chloe Branciforte
-
Figure 8.9: “Kanaga Volcano” (Public Domain; Michelle Combs/USGS via Flickr)
-
Figure 8.10: “Devils Postpile” (Public Domain; NPS)
-
Figure 8.11: “Collecting Gas Sample at a Fumarole” (Public Domain; Fred Murphy/USGS via Flickr)
-
Figure 8.12: “GVP-02668” (Public Domain; Harry Glicken/USGS)
-
Figure 8.13: "Volcanic Bomb" (Public Domain; Mark A. Wilson via Wikimedia Commons)
-
Figure 8.14: “Etna-Lapilli” (CC-BY-SA 3.0; Ji-Elle via Wikimedia Commons)
-
Figure 8.15: “Etna-Lapilli” (Public Domain; USGS)
-
Figure 8.16: Derivative of “Olympus Mons” (Public Domain; NASA) by Chloe Branciforte
-
Figure 8.17: “Amboy Crater” (Public Domain; Bob Wick/BLM via Fickr)
-
Figure 8.18: “Stratovolcano Cross-section” (CC-BY-SA 3.0; Woudloper via Wikimedia Commons)
-
Figure 8.19: “Volcano Sizes” (CC-BY 4.0; Chloe Branciforte, own work)
-
Table 8.2: “Volcano Types” (CC-BY 4.0; Chloe Branciforte, own work)
-
Figure 8.20: Derivative of “Yellowstone Hot Spot Track” (CC-BY 3.0; Kelvin Case/NPS via Wikimedia Commons) by Chloe Branciforte
-
Figure 8.21: Derivative of “Types of Volcanoes and Eruption Features” (CC-BY-SA 4.0; Chiara Cingottini, DensityDesign Research Lab via Wikimedia Commons) by Chloe Branciforte
-
Figure 8.22: “Volcanic Explosivity Index (VEI)” (Public Domain; USGS)
-
Figure 8.23: Derivative of “Living with Volcano Hazards” (Public Domain; Lisa M. Faust and Bobbie Myers, USGS) by Chloe Branciforte
-
Figure 8.24: “Volcanic Monitoring Types and Methods Employed by the USGS” (Public Domain; Lisa M. Faust, USGS)